1 Introduction
Calcareous soils are common in Tunisia [1,2]. Consequently, iron deficiency is among the major problems affecting species yield in the country [3,4]. Despite the abundance of this micronutrient in most soils, it is not readily available for roots of many plants, since it is mainly found as stable Fe(III) compounds with low solubility at alkaline pH [5,6]. To overcome this constraint, many plant species have evolved specific mechanisms to acquire this nutrient, which is essential for their metabolism. In fact, when subjected to Fe deficiency and in order to increase iron availability in the soil, dicots and non-graminaceous monocots induce some responses involving especially a plasma membrane H+-ATPase to increase root proton release capacity and a plasma membrane Fe(III) chelate reductase to reduce Fe(III) chelates at root surface (strategy I). It is well known that high bicarbonate content in these soils is the major factor responsible for the decrease of Fe(III) oxide solubility as well as the limitation of iron uptake by root cell cytosol [7,8]. In addition to the harmful effects of these anions () as a buffer neutralizing the proton release by root plasmalemma H+-ATPase [7], they also inhibit iron transport to shoots and its transfer from apoplasm to cytoplasm in shoot tissues [9,10].
Salinity is also one of the major factors that affect plant growth in Tunisia, where there is a wide variety of saline-sodic soils in depressions and in the main sebkhas and chotts [1]. Moreover, in irrigated areas, the awful quality of irrigation water charged with dissolved salts has resulted unfortunately in soil secondary salinization responsible for drops in productivity [1]. In saline and sodic soils, the solubility of micronutrients (e.g., Cu, Fe, Mn, Mo, and Zn) is particularly low, and plants grown on such soils often suffer from deficiencies in these elements [11]. However, relatively to the other micronutrients, few investigations studied salt influence on shoot iron concentration [12–14] and found contrasting results. Indeed, reductions were noticed in barley, corn [12], and pea [13], whereas enhancements were obtained in tomato, soybean, and squash [14]. Moreover, little is known about salinity interaction with iron deprivation. Indeed, as far as we know, the interaction between the two stresses was studied only by Gharsalli et al. [15] in sunflower (strategy I), and by Yousfi et al. [16] in barley (strategy II).
The aim of the present work was therefore to investigate interactive effects of salinity and iron deficiency on growth, chlorophyll content of young leaves, iron and potassium uptake, as well as root acidification in Medicago ciliaris L., a species abundant in saline ecosystems in Tunisia. To avoid any interference between (induced iron deficiency) and NaCl effects, we subjected plants to a direct iron deficiency.
2 Materials and methods
2.1 Generalities
Seeds of M. ciliaris were collected from the sebkha of Soliman (35 km northeast of Nabel, Tunisia) and sown in Petri dishes on filter paper moistened with distilled water. After three days, seedlings were beforehand grown during three weeks in liquid medium with a complete quarter-strength Hoagland's nutrient solution [17] in a growth chamber under controlled environmental conditions: a 14:10 h light/dark cycle, a 22:18 °C day/night temperature, a light intensity of 200 mmol m−2 s−1 PAR, and a relative humidity of 60–80%. The iron solution was prepared following Jacobson's method [18]. Thereafter, seedlings were divided into four lots and four treatments were started: C = control (complete medium (CM) containing 30 μM Fe), D = direct deficiency (CM containing only 1 μM Fe), S = salt treatment (CM containing 30 μM Fe with 75 mM NaCl), and DS = interactive treatment (CM containing 1 μM Fe and 75 mM NaCl). Nutrient solution (pH 6.0) was weekly renewed. During the first 14 days of treatment, a follow-up of the chlorosis index was carried out according to Gildersleeve and Occumpaugh [19]. Then, during the last eight days of treatment, a follow-up of the medium pH was realized. Values were determined every two days with a metrohm 633 pH-meter. Just before harvest, eight plants per treatment were incubated for three hours in a KCl (10 mM)/CaCl2 (1 mM) solution initially adjusted to pH 6.3, then proton extrusion fluxes were measured by a return titration using a NaOH solution (1 mM). Eight other plants per treatment were used to determine the total chlorophyll content of young leaves according to Torrecillas et al. [20] using the equation of Strain and Svec [21]. The remaining plants (8) were used for the determination of growth parameters and mineral composition (Na+, K+, and total Fe). On the 30th day of treatment, plants were harvested, separated into shoots and roots, weighed, dried at 60 °C, and then ground with an agate grinder.
2.2 Determination of the chlorosis index
The chlorosis index was determined in iron-deficient plants every two days following the scale of Gildersleeve and Occumpaugh [15]: (0) green young leaves, (1) slight chlorosis with specific yellow leaf margins, (2) yellow limb with green vascular bundles (3) completely yellow leaves, and (4) severe chlorosis with necrosis symptoms. A number was attributed to each plant according to the chlorotic state of its young leaves and, every two days, a mean of 24 replicates was calculated for each treatment.
2.3 Determination of chlorophyll content
A hundred milligrams of small discs from young leaves were incubated in 5 ml 80% acetone in darkness at 4 °C during three days (until complete chlorophyll extraction). Then the total chlorophyll content was determined by a lecture of absorbance at 649 and 665 nm and calculated using the equation of Strain and Svec [22]: total chlorophyll (μg ml−1) = 6.45 (A665) + 17.72 (A649).
2.4 Nutrient extraction and analysis
Mineral nutrients were extracted following the method described by Grusak (1995): samples from the ground vegetal matter were wet-digested in borosilicate tubes by adding a mixture of nitric and perchloric acids (3v:1v), heating at 150 °C for 1 h, and then heating at 200 °C until samples were near dryness. Blank and standards were run in parallel. Digests were thereafter dissolved in 1 ml of 2 M HNO3. Ion concentrations were determined by atomic absorption spectrophotometry (VARIAN 220 FS).
2.5 Statistical analysis
Variance analysis of data (ONE-WAY ANOVA) was performed using the SPSS 10.0 program, and means were separated according to Duncan's test at .
3 Results
3.1 Biomass production in shoots and roots
Both salinity and iron deficiency resulted in a significant decrease in plant growth (Fig. 1). Nevertheless, the effect of the former was more pronounced than that of the latter in shoots as well as in roots. In addition, we found that iron deprivation had a similar effect on both organs (54% of the control dry weight), whereas salinity affected more roots (34%) than shoots (22%). Under the interactive effects of the two constraints, we registered a considerable decline in plant growth affecting at the same degree shoots and roots (80%), and indicating that the two individual effects were additive.
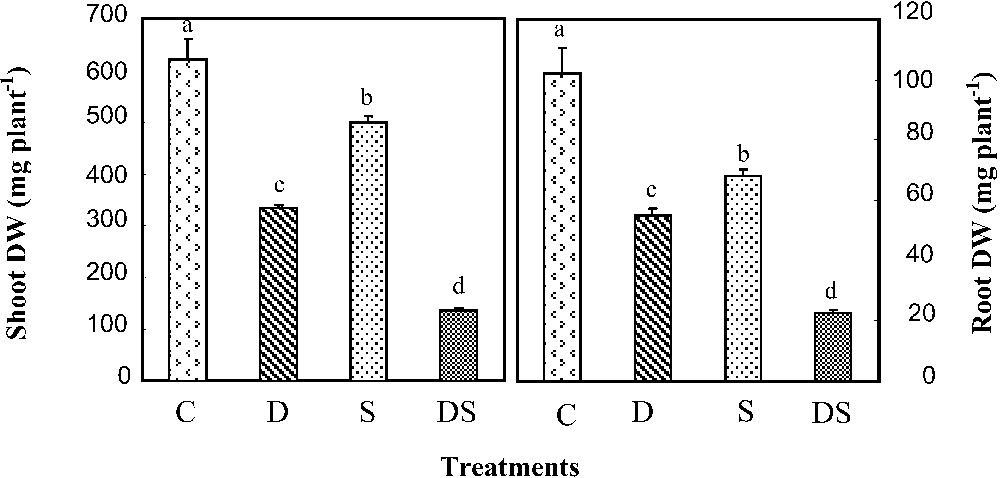
Shoot and root dry weights in M. ciliaris plants grown during 14 days on a control nutrient solution (C), containing only 1 μM Fe (D), with 75 mM NaCl (S), or under interactive effects of salinity and iron deficiency (DS). Values are means of eight replicates ± standard error. Bars with different letters are different according to the ANOVA test at P⩽0.05.
3.2 Chlorosis index and chlorophyll content
The chlorosis index increased slowly during the first 10 days, then was stabilized at about 0.5 and 0.7, respectively, in D and DS plants. At the end of the follow-up, the chlorophyll content was about 2.3 mg g−1 FW. It was reduced by 22% under iron deficiency conditions (D) and displayed no significant variation in the salt medium (S). Under interactive conditions, a more pronounced effect on the chlorophyll content (56% of the control) was observed, indicating an addition of the two individual effects to each other.
3.3 Sodium, potassium, and iron acquisition
3.3.1 Sodium
Plants subjected only to salt stress accumulated more than 1.2 mmol Na+ g−1 DW in their shoots, against less than 0.6 mmol Na+ g−1 DW in their roots (Figs. 2 and 3). This behaviour led to the accumulation of high sodium quantities in the former, exceeding 15 times those accumulated in the latter. Under interactive effects, statistically significant variations in Na contents were observed: it increased in shoots and decreased in roots. Quantities, however, were diminished in both plant parts.

(A) Chlorosis index according to Gildersleeve and Occumpaugh's (1989) scale in young leaves of M. ciliaris plants grown during 14 days on a nutrient solution containing only 1 μM Fe added (DS) or not (D) with 75 mM NaCl. Values are means of 24 replicates ± standard error. (B) Chlorophyll content of the young leaves of M. ciliaris plants grown during 14 days on a control nutrient solution (C), containing only 1 μM Fe (D), with 75 mM NaCl (S), or under interactive effects of salinity and iron deficiency (DS). Values are means of eight replicates ± standard error. Bars with different letters are different according to the ANOVA test at P⩽0.05.

Na+ contents and quantities in shoots and roots of M. ciliaris plants grown during one month on a control nutrient solution (C), containing only 1 μM Fe (D), with 75 mM NaCl (S), or under interactive effects of salinity and iron deficiency (DS). Values are means of eight replicates ± standard error. Bars with different letters are different according to the ANOVA test at P⩽0.05.
3.3.2 Potassium
Control plants displayed a potassium content of about 0.7 mmol K+ g−1 DW in their shoots and a content of 0.9 mmol K+ g−1 in their roots. Under iron starvation conditions, no significant effect on the K content was observed in shoots, whereas the root K content was reduced by more than 40% (Fig. 4). Its quantity was restricted in shoots, but more affected in roots. This stress was indeed able to decrease the potassium absorption efficiency (KAE) by 25% (Fig. 5). In salt-stressed plants, both K content and quantity showed a strong decline in all organs, mainly in roots, in which they respectively represented 14 and 8% of the control. An enormous restriction was also observed in their root KAE, which was reduced by 75%. In plants subjected to interactive effects, we did not find any difference in K+ content with those grown under salinity. However, the K quantity was drastically reduced in all their organs. In these plants, root KAE was less than 4% of the control. As a response to such a situation, plants increased their shoot potassium use efficiency (KUE) (Fig. 5). The highest value (five times the control) was obtained in DS plants.

K+ contents and quantities in shoots and roots of M. ciliaris plants grown during one month on a control nutrient solution (C), containing only 1 μM Fe (D), with 75 mM NaCl (S), or under interactive effects of salinity and iron deficiency (DS). Values are means of eight replicates ± standard error. Bars with different letters are different according to the ANOVA test at P⩽0.05.
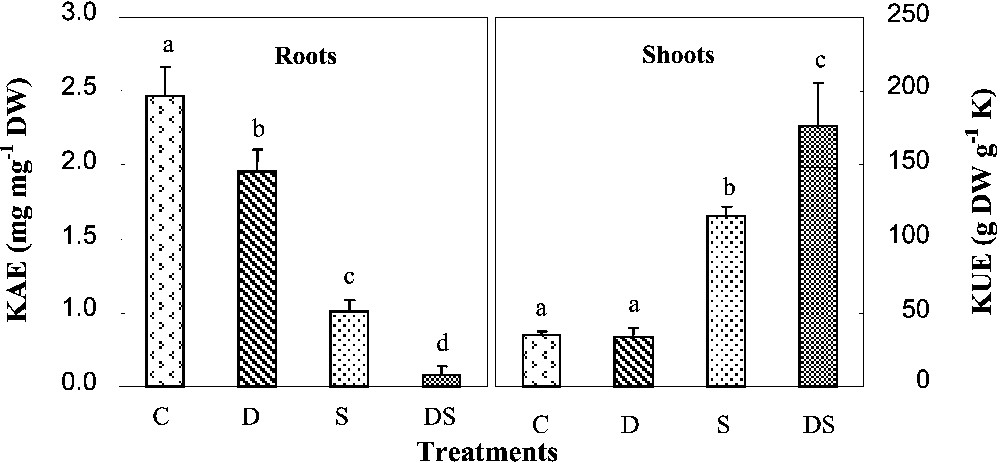
Potassium absorption (KAE) and use (KUE) efficiencies in M. ciliaris plants grown during one month on a control nutrient solution (C), containing only 1 μM Fe (D), with 75 mM NaCl (S), or under interactive effects of salinity and iron deficiency (DS). Values are means of eight replicates ± standard error. Bars with different letters are different according to the ANOVA test at P⩽0.05. The potassium absorption efficiency (KAE) was determined as the ratio of total K+ quantity accumulated in each plant during the experiment to its root dry weight. The potassium use efficiency (KUE) was calculated as the ratio of shoot biomass production in each plant during the experiment to the quantity of K+ accumulated in these organs during the same period.
3.3.3 Iron
Our results indicated that iron was more or less equally distributed within the control plant. In addition, when cultivated during one month under iron starvation conditions, D and DS plants showed a significant decline of iron contents in all organs (Fig. 6). Quantities of this nutrient were drastically decreased, especially in plants grown under interactive effects of the two stresses. In such plants, they represented in each organ about 21% as compared to the control. Less pronounced decreases in Fe contents were also obtained in salt-stressed plants, in which they were diminished by 46 and 38% respectively in shoots and roots, which corresponded to a diminution of nearly 50% of the Fe quantities in both plant parts. In all treated plants (treatments S, D, and DS), iron absorption efficiency (FeAE) was reduced (Fig. 7). The lowest value was observed in DS plants, in which it represented less than 4% of the control. Under such severe conditions, plants increased their shoot iron use efficiency (FeUE), reaching in DS plants six times those of the control (Fig. 7).

Total iron contents and quantities in shoots and roots of M. ciliaris plants grown during one month on a control nutrient solution (C), containing only 1 μM Fe (D), with 75 mM NaCl (S), or under interactive effects of salinity and iron deficiency (DS). Values are means of eight replicates ± standard error. Bars with different letters are different according to the ANOVA test at P⩽0.05.
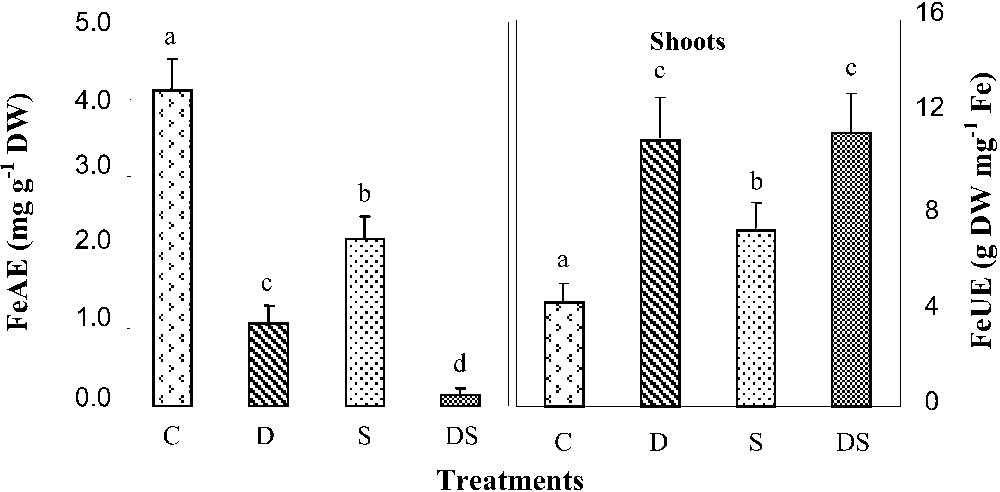
Iron absorption (FeAE) and shoot use (FeUE) efficiencies in M. ciliaris plants grown during one month on a control nutrient solution (C), containing only 1 μM Fe (D), with 75 mM NaCl (S), or under interactive effects of salinity and iron deficiency (DS). Values are means of eight replicates ± standard error. Bars with different letters are different according to the ANOVA test at P⩽0.05. Iron absorption efficiency (KAE) was determined as the ratio of total Fe quantity accumulated in each plant during the experiment to its root dry weight. The iron use efficiency (KUE) was calculated as the ratio of the shoot biomass production in each plant during the experiment to the quantity of Fe accumulated in these organs during the same period.
3.4 Root proton release
The pH of the nutrient solution was measured every two days during the last eight days of treatment and values were represented in Fig. 8A. In control plants, the medium pH values, initially adjusted to pH 6, tended to increase and exceeded 7, whereas roots of plants grown under iron starvation showed an important acidification of the nutrient solution. Indeed, D plants showed the lowest pH value on the fourth day of measurement. This response to iron deficiency was reduced under salt stress in plants subjected to the interactive effects.

pH values of the culture media during the last eight days of treatment (A) and proton release rate (PRR) as measured after a 3-h incubation in a KCl (10 mM)/CaCl2 (1 mM) medium just before the harvest (B) in M. ciliaris plants grown during one month on a control nutrient solution (C), containing only 1 μM Fe (D), with 75 mM NaCl (S), or under interactive effects of salinity and iron deficiency (DS). Values are means of eight replicates ± standard error. Bars with different letters are different according to the ANOVA test at P⩽0.05.
We also measured the root acidification capacity on a KCl/CaCl2 medium in order to compare clearly the ability of plants subjected to each treatment to extrude protons by their root epidermis cells (Fig. 8B). Results showed a high proton release in plants grown under iron starvation, which was drastically inhibited by salt. The proton release rate of plants subjected to the interactive effects was reduced by more than 65% as compared to those subjected to iron deficiency. Nevertheless, the depressive effect of salinity as applied alone on the proton release rate was not statistically significant in S plants.
4 Discussion
M. ciliaris was a relative salt-tolerant species, as a 75-mM-NaCl treatment did not severely affect its growth (Fig. 1). These results are in agreement with those of Abdelly et al. [23] who worked on a more tolerant species from Tunisia (originating from Enfidha, central-eastern Tunisia) and found no growth restriction at 110 mM NaCl. M. ciliaris appeared also as a sensitive plant to direct iron deficiency, since it lost about 50% of its dry weight under this stress (Fig. 1). Indeed, several physiological and biochemical activities are iron-dependent [24,25]. In DS plants, the effects of the two stresses on biomass production were additive (Fig. 1). Similar results were described by Gharsalli et al. [15] and Yousfi et al. [16], who noticed that the interaction of the two stresses led to a marked growth decline respectively in sunflower and barley. Gharsalli et al. [15] demonstrated also that this interactive effect increased with increasing salinity.
The chlorophyll content of young leaves was also affected especially by iron starvation, which explained the appearance of iron chlorosis symptoms (Fig. 2) often described in Fe-deficient plants [3,4,26]. This micronutrient is necessary for chlorophyll biosynthesis as well as thylakoïd and granum formation [27–29]. Consequently, iron chlorosis is due to chlorophyll dilution when leaves continue to grow at a normal rate under iron deficiency conditions [30] and to cell inability to produce and/or to stabilize new chlorophyll molecules in thylakoïd membrane [31]. Our results revealed that this dilution phenomenon occurred in D plants despite the great decline in their leaf expansion (not shown). The effect was much more pronounced in DS plants, in which young leaf chlorophyll content was reduced by 44% in comparison with the control. This suggested a more pronounced reduction of chlorophyll biosynthesis and/or a stronger stimulation of their biodegradation. In fact, although salinity did not significantly affect chlorophyll content when applied separately, it seemed to accentuate the harmful effect of iron starvation on the content of these pigments in DS plants. However, salinity was often shown to decrease chlorophyll content in photosynthetic organs, as reported in other plants (e.g., [32]).
Salt stress severely affected iron transport to shoots, since its content was more reduced in aerial organs than in roots (Fig. 6). Shiyab et al. [33] explained such a salt-induced decrease in iron transport that they found in in-vitro-grown shoots of sour orange by an antagonism between Na and Fe. Salinity was also shown to reduce Fe content in shoots of barley, corn [14], and pea [12]. However, it enhanced it in tomato, soybean, and squash [13]. In fact, as for all micronutrients, the relationship between salinity and iron is complex, and salt stress may increase, decrease, or have no effect on Fe content in plant shoots. Differences can be attributed to plant type and tissue, salinity level and composition, iron concentration in the medium, growing conditions, and the duration of study [34].
In addition to sodium effects on shoot growth, chlorophyll content, and iron transport, it affected, at the root level, both growth and nutrient acquisition. Potassium uptake was drastically inhibited; its absorption efficiency was reduced by 75%. This reduction has been often associated with a sodium antagonist effect [35–37]. Potassium influx transporters were indeed shown to mediate sodium influx into root cells. These transporters belong at least to two different mechanisms: a high-affinity uptake system saturable at the micromolar range and a low-affinity uptake system saturable at the millimolar range [38]. In higher plants, potassium homeostasis is maintained by several protein families serving as K+ channels and transporters [39]. A Na+-coupled high-affinity K+ system was shown to be the major form of high-affinity K+ uptake in numerous species [40]. In wheat, extracellular sodium concentration is responsible for the stimulation of HKT1 high-affinity K+ uptake at the micromolar range and its blockage at highly toxic concentrations [41]. Working on OsAKT1 (AKT1-type potassium channel transcripts) transcription in three rice lines differing in their tolerance to salt stress, Golldack et al. [42] showed that “in the salt-tolerant, sodium-excluding varieties Pokkali and BK, OsAKT1, transcription disappeared from the exodermis in plants treated with 150 mM NaCl for 48 h, but OsAKT1 transcription was not repressed in these cells in the salt-sensitive, sodium-accumulating variety IR29”. They noticed that this was correlated with the overall tolerance in the species.
A decrease in potassium acquisition was also observed in D plants. Indeed, iron starvation is often associated with potassium starvation, although some authors suppose that chlorotic organs frequently exhibit high K+ contents [24]. Consequently, in plants subjected to the interactive effects, potassium acquisition was severely inhibited in comparison with those of plants cultivated under salinity or iron deficiency when applied separately (Fig. 5). In fact, our results showed that KAE was decreased by 25% in plants treated only with Fe deficiency, by 75% in plants subjected to salt alone, and by more than 95% in plants exposed simultaneously to salinity and Fe deficiency. Therefore, our results suggest that both stresses affected K+ transport systems.
Iron acquisition was also affected by salt stress; Fe absorption efficiency was reduced by 25% in S plants (Fig. 7). This limitation could be due to salt effect on iron transporters and/or on Fe(II)-chelate reductase, since the salt inhibitory effect on the root acidification capacity was not statistically significant. Salt effect on iron uptake was more pronounced in DS than in S plants (Fig. 7). The difference could be due to the inability of the formers to take up iron traces from the saline medium. Indeed, our results showed that root acidification was significantly reduced in plants subjected simultaneously to salt and iron deficiency relatively to those treated with Fe deficiency alone. In the same way, an inhibitory effect of salinity on physiological responses of strategy-II plants to iron deficiency was described by Yousfi et al. [16], who found an inhibition of phytosiderophore release in barley under saline conditions.
Nutrient uptake involves a root plasma membrane H+-ATPase; the electrochemical potential it generates serves as a driving force for the transmembrane flux of other ions by uniport, H+-symport, and H+-antiport mechanisms [43]. Under iron starvation, a stimulation of the root plasma membrane H+-ATPase has been often reported in strategy-I plants [3,4,26], and is considered together with the stimulation of a Fe(III)-chelate reductase as the key physiological responses to iron deficiency in these plants [5,6]. However, salt influence on root proton pump is an issue of several controversies; salinity decreased plasma membrane H+-ATPase activity in wheat [44], but improved it in Vigna radiata [45] and Citrus sinensis [46]. In tomato, the activity of this pump was shown to decrease [47] or to have no response [48] to salt stress, depending probably on varieties. Some studies on plasma membrane and vacuolar H+-ATPases in plant leaves showed an enhancement of their activities under saline conditions [49]. Such stimulation was even considered as involved in salt adaptation of tomato calli [50] and Mesembryenthemum crystallinum L. cell suspensions [51,52], and whole plants [49]. Furthermore, Debez et al. [53] demonstrated that salt tolerance in the halophyte Cakile maritima involved two different leaf proton pumps: an increase of vacuolar H+-ATPase activity was observed up to 300 mM NaCl and a stimulation of plasma membrane H+-ATPase was detected at the intense salinities. Nevertheless, taking in account the few works that demonstrated salt-induced activities of these pumps, it is not yet possible to draw firm conclusions on the value of their activities as indicators of salt tolerance [54].
Our results showed that: (i) Fe deficiency increased proton release, (ii) the decrease of proton release rate by salinity in S plants was not statistically significant, (iii) salt and Fe deficiency when applied simultaneously resulted in an intermediate acidification capacity. These data supported the hypothesis of Gharsalli and Hajji [55], who suggested the existence of two plasma-membrane proton pumps: one for the basic acidification and another for iron deficiency-induced acidification, which can be inhibited by salt stress. In addition, Gharsalli et al. [15] demonstrated that this effect increased with NaCl concentration and treatment period.
In conclusion, direct iron deficiency resulted in a decline in biomass production and young leaf chlorophyll content in M. ciliaris. Nevertheless, iron-deficient plants responded to the stress by a great root acidification capacity. In this species, the significant inability of salt-treated plants to prevent their photosynthetic organs from sodium invasion could explain the marked decline of their growth. Moreover, salt stress not only restricted macronutrient acquisition, but also limited the uptake of micronutrients like iron. As applied simultaneously with iron deficiency, salinity limited the aptitude of the species to take up Fe traces from the medium. This fact was probably due to salt influence on root acidification, since under iron starvation conditions, this dicot showed a marked increase in its root proton-release capacity, with the aim to improve iron availability in the medium.
Acknowledgements
The authors are grateful to the grammatical overseer of the manuscript, Dr. Nebil Cherni, English Department, Faculty of Letters, Arts, and Humanities of Manouba, University of Manouba.