1 Introduction
Cellular damage or oxidative injury arising from free radicals or reactive oxygen species (ROS) now appears the fundamental mechanism underlying a number of human diseases [1]. Antioxidants are radical scavengers, which protect the human body against free radicals that may cause pathological conditions such as anaemia, asthma, arthrists, inflammation, neurodegeneration, Parkinson's diseases, mongolism, ageing process, and perhaps dementias [2]. Free radicals can be scavenged through chemoprevention utilizing natural antioxidant compounds present in foods [3] and medicinal plants [4]. Some medicinal plants have been shown to have both chemopreventive and/or therapeutic effects on human diseases [5].
A pathway for ascorbic acid (AA) biosynthesis featuring GDP-mannose and l-galactose has recently been proposed for plants. AA is a very important reducing substrate for H2O2 detoxification in photosynthetic organisms [6]. AA participates in the removal of H2O2 as a substrate of ascorbate peroxidase (APX). Reduced glutathione (GSH) is another most important non-enzymatic antioxidant molecule, which functions as an effective ROS detoxifier [7]. α-Tocopherol (α-toc) was consumed predominantly as a radical scavenging antioxidant against lipid peroxidation [8].
The enzymatic antioxidant defence system includes superoxide dismutase (SOD), ascorbate peroxidase (APX), polyphenol oxidase (PPO), catalase (CAT) and glutathione reductase (GR) [9]. SOD is a major scavenger of superoxide anion radical () that catalyses the dismutations of with great efficiency, resulting in the production of H2O2 and O2 [10]. The H2O2 scavenging system represented by APX and CAT are more important in imparting tolerance than SOD, as reported in plants subjected to oxidative sreesses [11]. PPO is believed to be ubiquitous in the plant kingdom and they are primarily associated with the enzymatic browning and off-flavour generation [12].
White yam (Dioscorea rotundata Poir.) is one of the important edible tuber crops cultivated in India and many other tropical countries, including Africa [13]. Yams are valuable sources of carbohydrate, fibres, and low-level fats, which makes them a good dietary nutrient, and also processed into various staple, intermediate, and end-product forms [14]. Yams also have medicinal properties. Several species of Dioscorea are amongst the principle sources of diosgenin, which can be converted into medicinally important steroids [15]. Diosgenin is transferred to serum dehydroepiandrosterone in human intestine and is associated with reduced lipid peroxidation and lowered serum triglycerides [16]. A lot of works have already covered the food value [17], nutritional aspects [18], cultivation [19], processing technologies [20], medicinal aspects [21,22], growth-regulator effects [14,23,24], tissue culture, and genetic stability studies [25] of yam plants. But only little attention has been drawn to the antioxidant properties of this food plant. Although there are reports on effects of temperature on antioxidative status of yams [26] and antioxidant variations in different physiological regions of yam tubes [12], the literature regarding antioxidant properties and methods to enhance the antioxidant potentials in white yam is scarce. It therefore seems important to test the methods to increase the innate antioxidant potential of this food crop, in order to satisfy the needs for antioxidants in diet and thereby make it an economically important food crop.
Triazoles are a group of compounds, which have both fungitoxic and plant growth-regulating properties [27]. In addition, they can also protect plants against various environmental stresses [28]. Triazoles affect the isoprenoid pathway, and alter the levels of certain plant hormones by inhibiting gibberellin synthesis, reducing ethylene evolution, and increasing cytokinin levels [29]. Some of the previous works carried out in our lab revealed the morphological and physiological changes associated with triazole treatment in various plants, including inhibition of plant growth, decreased internodal elongation, increased chlorophyll levels, enlarged chloroplasts, thicker leaf tissue, increased root-to-shoot ratio, alkaloid production and enhancement in carbohydrate metabolism [14,24,30–38]. Paclobutrazol (PBZ) [(2RS; 3RS)-1-(4-chlorophenyl)-4,4-dimethyl-2-(1H-12,4-triazol-1-yl)-pentan-3-ol], a triazole, fungicide, having plant-growth regulator (PGR) properties, is reported to inhibit gibberellic acid (GA) biosynthesis and increase abscisic acid (ABA) and cytokinin contents [27]. Therefore, there is a need to investigate the efficacy of this compound in the enhancement of antioxidant potentials in white yam plants in order to increase their medicinal properties, thereby making them an economically valuable crop plant. Hence, this study aims to evaluate the ability of PBZ to enhance the antioxidative potentials, with special emphasis to both non-enzymatic and enzymatic antioxidant constituents.
2 Materials and methods
2.1 Plant materials and PBZ treatment
Tubers of Dioscorea rotundata cv. Sree Priya were obtained from the Central Tuber Crop Research Institute (CTCRI), Kerala, India, and planted at the Botanical Garden of the Annamalai University. PBZ was obtained as CULTAR 25% w/v. In the preliminary experiments, four concentrations (10, 15, 20, and 25 mg l−1 plant−1) of PBZ were prepared and used for tuber treatment, in order to determine the optimum concentration at which maximum sprouting occurred. Among these concentrations, 15 mg l−1 plant−1 was found to enhance the antioxidant potentials; at lower (10 mg l−1 plant−1) and higher (20 and 25 mg l−1 plant−1) concentrations, there was no significant effect. Hence, 15 mg l−1 plant−1 were used for this study. The treatment was given by soil drenching on three vegetative growth periods, i.e., 30, 60, and 90 DAP. For each treatment, one litre of the solution was used. The control plants were given tap water. The plants were uprooted on 100 DAP washed and separated into leaf stem and tubers for extraction and assay of antioxidant potentials.
2.2 Non-enzymatic antioxidant estimations
2.2.1 Ascorbic acid content
Ascorbic acid (AA) content was assayed as described by Omay et al. [39]. The extract was prepared by grinding 1 g of fresh material with 5 ml of 10% TCA, centrifuged at 3500 rpm for 20 min, and re-extracted twice; the supernatant was made up to 10 ml and used for assay. To 0.5 ml of extract, 1 ml of DTC reagent (2,4-dinitrophenyl hydrazine-thiourea-CuSO4 reagent) was added, incubated at 37 °C for 3 h and 0.75 ml of ice-cold 65% H2SO4 was added, allowed to stand at 30 °C for 30 min; the resulting colour was read at 520 nm in a spectrophotometer (U-2001-Hitachi). The AA content was determined using a standard curve prepared with AA.
2.2.2 Reduced glutathione
The reduced glutathione (GSH) content was assayed as described by Griffith and Meister [40]. 200 mg of fresh material was ground with 2 ml of 2% metaphosphoric acid, and centrifuged at 17,000 rpm for 10 min. The supernatant was neutralized by adding 0.6 ml of 10% sodium citrate. 1 ml of assay mixture was prepared by adding 100 μl of extract, 100 μl of distilled water, 100 μl of 5,5-dithio-bis-(2-nitrobenzoic acid), and 700 μl of NADPH. The mixture was stabilized at 25 °C for 3–4 min. Then 10 μl of glutathione reductase were added, and the absorbance was read at 412 nm in a spectrophotometer.
2.2.3 α-Tocopherol content
α-Tocopherol (α-toc) activity was assayed as described by Backer et al. [41]. 500 mg of fresh tissue were homogenized with 10 ml of a mixture of petroleum ether and ethanol (2:1.6 v/v), and the extract was centrifuged at 10,000 rpm for 20 min, whereas the supernatant was used for estimation of α-toc. To 1 ml of extract, 0.2 ml of 2% 2,2-dipyridyl in ethanol was added and mixed thoroughly and kept in dark for 5 min. The resulting red colour was diluted with 4 ml of distilled water and mixed well. The resulting colour in the aqueous layer was measured at 520 nm. The α-toc content was calculated using a standard graph made with known amount of this species.
2.3 Antioxidant enzyme extractions and assays
2.3.1 Superoxide dismutase (SOD, EC 1.15.1.1)
The activity of SOD was assayed as described by Beauchamp and Fridovich [42]. The reaction mixture contained of riboflavin, 0.1 M of methionine, of KCN, and of nitroblue tetrazolium (NBT) salt dissolved in 3 ml of 0.05 M sodium phosphate buffer (pH 7.8). Three millilitres of the reaction medium were added to 1 ml of enzyme extract. The mixtures were illuminated in glass test tubes by two sets of Philips 40-W fluorescent tubes in a single row. Illumination was started to initiate the reaction at 30 °C for 1 h. Identical solutions that were kept under dark served as blanks. The absorbance was read at 560 nm in the spectrophotometer against the blank. The SOD activity is expressed in U mg−1 protein (U = change in 0.1 absorbance h−1 mg−1 protein).
2.3.2 Ascorbate peroxidase (APX, EC 1.11.1.1)
The activity of APX was determined by the method of Asada and Takahashi [43]. The reaction mixture (1 ml) contained 50 mM of potassium phosphate buffer (pH 7.0), 0.5 mM of ascorbic acid, 0.1 mM H2O2, and 200 μl of enzyme extract. The absorbance was read as decrease at 290 nm against the blank, correction was done for the low, non-enzymatic oxidation of ascorbic acid by H2O2 (extinction coefficient 2.9 mM−1 cm−1). The enzyme activity was expressed in units mg−1 protein (U = change in 0.1 absorbance min−1 mg−1 protein).
2.3.3 Polyphenol oxidase (PPO, EC 1.10.3.1)
The assay of PPO was carried out by the method of Kumar and Khan [44]. Assay mixture for PPO contained 2 ml of 0.1 M phosphate buffer (pH 6.0), 1 ml of 0.1 M catechol, and 0.5 ml of enzyme extract. This was incubated for 5 min at 25 °C, after which the reaction was stopped by adding 1 ml of 2.5 N H2SO4. The absorbance of the purpurogallin formed was read at 495 nm. 2.5 N H2SO4 was added at the zero time to the blank of the same assay mixture. PPO activity is expressed in U mg−1 protein (U = change in 0.1 absorbance min−1 mg−1 protein).
2.3.4 Catalase (CAT, 1.11.1.6)
The activity of CAT was measured according the method of Chandlee and Scandalios [45] with small modifications. The assay mixture contained 2.6 ml of 50 mM potassium phosphate buffer (pH 7.0), 0.4 ml of 15 mM H2O2, and 0.04 ml of enzyme extract. The decomposition of H2O2 was followed by the decline in absorbance at 240 nm. The enzyme activity was expressed in units mg−1 protein (U = 1 mM of H2O2 reduction min−1 mg−1 protein). The enzyme protein was estimated by the method of Bradford [46] for all the enzymes.
2.4 Statistical analysis
Statistical analysis was performed using the one-way analysis of variance (ANOVA) followed by the Duncan's Multiple Range Test (DMRT). The values are mean ± SD for six samples in each group. p values ⩽0.05 were considered as significant.
3 Results and discussion
The effect of PBZ treatments on antioxidant potentials was previously checked after seven days of each treatment (data not shown) and it was shown that 90 DAP treatment and 100 DAP sampling were sufficient to indicate, in a reliable way, the new activity levels of the antioxidative status.
The non-enzymatic antioxidant, AA, content varied with the application of PBZ @ 15 mg l−1 plant−1 in D. rotundata plants when compared to control. Fig. 1a shows the changes in AA content of white yam on 100 DAP due to PBZ treatment. The AA content in leaf, stem, and tuber increased largely in PBZ-treated plants when compared to control. GSH content was highly influenced in all parts of the plants due to PBZ treatment (Fig. 1b). α-Toc content also increased under PBZ treatment in white yam when compared to control plants (Fig. 1c). The increase extent was larger in the tubers compared to other parts of the plant.
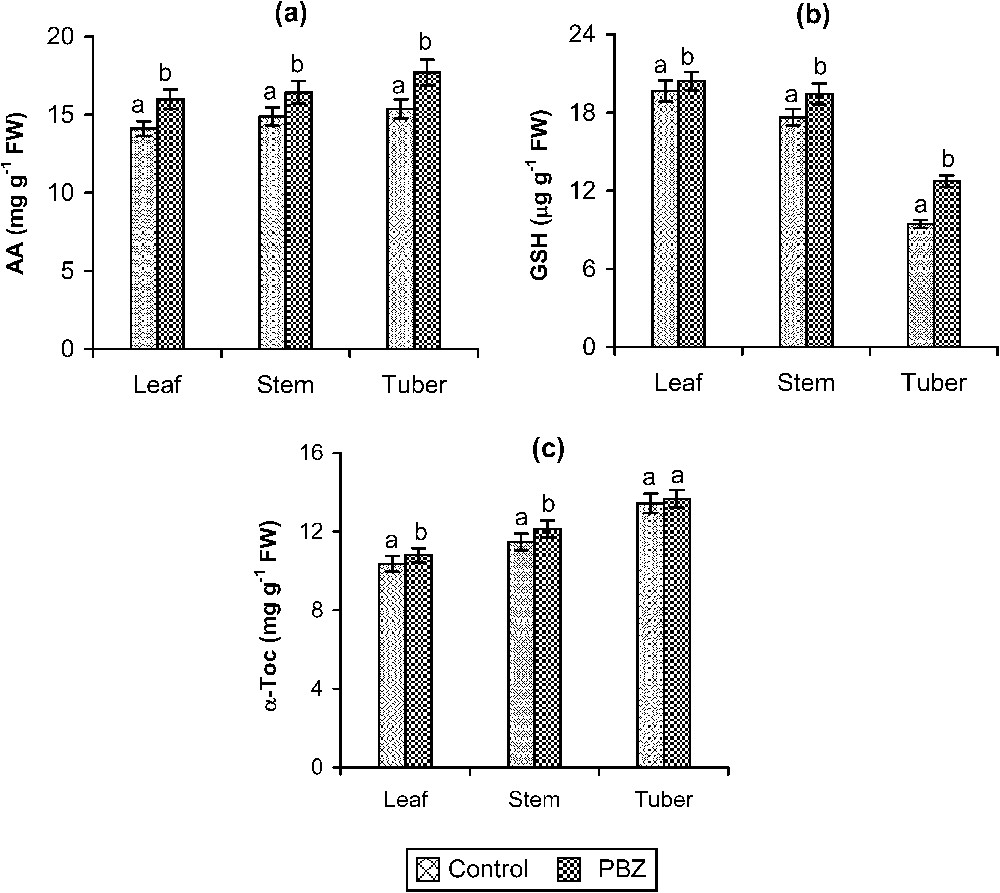
Effect of PBZ (15 mg l−1 plant−1) on AA (a), GSH (b) and α-toc (c) contents of leaf, stem, and tubers of Dioscorea rotundata plants. Values are given as mean ± SD of six samples in each group. Bar values are not sharing a common superscript (a, b) differ significantly at p⩽0.05 (DMRT).
The non-enzymatic antioxidant contents play major roles in maintaining the balance between free-radical production and elimination [47]. Jaleel et al. [48] have shown that plant-growth regulator treatments in Catharanthus roseus induced several changes in the antioxidative system profiles. In plants, the AA-glutathione cycle has been shown to be of great importance in free-radical scavenging and in multiple-stress reactions [49]. The enhancement of antioxidant defence mechanism under triazole treatment can be correlated with the ability of triazoles in protecting plants from abiotic stresses [33–35,37]. PBZ-treated plants have a more efficient free-radical scavenging system that enables them to detoxify active oxygen species [50]. The application of this triazole compound, being a fungicide that can alter the metabolic equilibrium, can exhibit stress-like symptoms in plants [51], but simultaneously it can protect plants from apparently unrelated abiotic stresses, like the NaCl one [34].
The increase in non-enzymatic antioxidants can be correlated with plants' native protective mechanism against oxidative stress arisen from fungicide application [36]. Being the major antioxidant species in plants, the contents in AA, GSH, and α-toc vary in different subcellular compartments, according to the intensity of the stress [52]. The GSH content was found increased under pesticide and herbicide application [53], as well as in drought-stressed Catharanthus roseus [47]. The increase in GSH can be correlated with its ability to scavenge single oxygen, peroxides, and hydroxyl radicals and is involved in recycling of AA in the ascorbate-glutathione pathway in chloroplasts [54]. An increase in the AA content was reported in Catharanthus plants under salinity [34,55]. AA being a triazole compound, our results are also in agreement with this. AA can function as the ‘terminal antioxidant’ on plants because the redox potential of ascorbate/monodehydro ascorbate pair (+280 nm) is lower than that of most of the bioradicals [56]. From out results, it can be concluded that the PBZ application can enhance largely the non-enzymatic antioxidants' quantity, which is of great importance in imparting economic values to the plant.
Fig. 2a represents the comparison of the effects of PBZ-treatment on SOD activity in leaf, stem, and tubers of white yam on 100 DAP with untreated control plants. In case of non-PBZ plants, the SOD-activity remains low, whereas the addition of PBZ enhanced the SOD-activity; this was highly marked over the control plants. Fig. 2b illustrates the APX activity in the yam plants under cultivation with or without PBZ-treatment on 100 DAP. From the figure, it is clear that the APX activity was increased due to PBZ treatment in all parts of the plant. In different parts (leaf, stem, and tuber), the PPO activity varied considerably. PPO activity increased due to PBZ treatment when compared to control on 100 DAP (Fig. 2c). The CAT activity was comparatively low in white yam plants. There was only a slight increase in the activity of this antioxidant enzyme upon treatment with PBZ (Fig. 2d).

Effect of PBZ (15 mg l−1 plant−1) on SOD (a), APX (b), PPO (c) and CAT (d) activities of leaf, stem, and tubers of Dioscorea rotundata plants. Values are given as mean ± SD of six samples in each group. Bar values are not sharing a common superscript (a, b) differ significantly at p⩽0.05 (DMRT).
Plants receiving PBZ have significantly higher antioxidative system levels when compared to plants under non-PBZ normal conditions [57]. The involvement of the antioxidative system in the regulation of free-radical metabolism was followed by measuring changes in the antioxidant enzyme activities when plants experience stress from an abiotic factor like a fungicide (PBZ); there should be an enhancement in the production of toxic free-radicals of H2O2, , •O2 or •OH, which should be detoxified in terms of increased antioxidant enzyme activities. Enhancement of SOD activity under PBZ treatment may be an indicator of superoxide production. High levels of SOD should be followed by scavenging of H2O2 catalysed by APX and CAT [47]. The APX found in organelles is believed to scavenge H2O2 produced from the organelles, whereas the function of cytosolic APX is probably to eliminate H2O2 that is produced in the cytosol or apoplast and that has diffused from organelles [58]. Increased APX, SOD, and CAT activities were reported in propiconazole-treated Vigna plants [33] and in Catharanthus roseus under drought stress [47]. The increase in CAT found under PBZ treatment was of great importance in plants' protective mechanisms under abiotic stress. The H2O2 scavenging system represented by CAT is more important in imparting tolerance than SOD, as reported in oxidative-stressed Catharanthus plants [59]. The changes in CAT may vary according to the intensity of stress, time of assay after stress and induction of new isozyme(s) [60]. The level of antioxidative response depends on species, the development, and the metabolic state of the plant, as well as on the duration and intensity of the stress [61]. In conclusion, our results indicated that the PBZ application at low concentration could be used as a potential tool to increase non-enzymatic and enzymatic antioxidant potentials in food plants like D. rotundata.