Abbreviations
SAM
shoot apical meristem
IAAindole-3-acetic-acid
PINpin-formed
LFYLEAFY
ANTAINTEGUMENTA
CUCCUP-SHAPED COTYLEDONS
NPAnaphthylpthalamic acid
LAXLike AUX1
PIDpinoid
PLTplethora
1 Introduction
In higher plants, post-embryonic development is characterized by the continuous production of tissues and organs during the whole plant life. Histogenesis and organogenesis involve apical zones of cells established during embryogenesis at the opposite poles of the plant embryo which are called meristems. The mitotic activity of pluripotent stem cells located in those meristems permits organ initiation. By comparison with animal stem cells, plant meristems are now considered as stem cell niches, i.e. cellular microenvironments that provide the signals and physical support to maintain stem cells [1]. Consequently, plant development depends on the organization, localization and maintenance of those stem cell niches, which involve several regulatory mechanisms including hormone signaling. The hormone auxin, from the Greek word “auxo” to increase (grow), induces various growth responses in plants. It has been identified at the end of the xix century by the Darwins studying the phototropism of canary grass coleoptiles. Auxin is implied in many developmental processes by inducing cellular and molecular modifications such as cell expansion, cell proliferation or transcription. In this short review, we will focus on the role of auxin in organogenesis at the SAM. Hereby, we will focus on the angiosperms, which have been best characterised.
2 Organogenesis at the SAM
The SAM produces lateral organs, such as leaves, and a growing stem. The SAM is a multilayered structure composed of up to three (sometimes even more) clonally distinct layers of cells, L1 to L3. The descendants of the L1 cells preferentially generate the epidermis, L2 cells produce sub-epidermal tissues and gametes whereas the L3 cell lineage will form inner stem tissues and lateral organs (for a review, see [2]). Organogenesis relies on spatially and temporally coordinated cell proliferation in the SAM. Differences in mitotic activities underline the existence of three cytological zones in the SAM which overlap the layered partitioning [3,4]: the peripheral zone on the sides, the central zone at the apex and the rib zone formed by the internal cells beneath the apex. These zones have different functions. Organ initiation takes place in the peripheral zone and the internal tissues of the stem (pith and vascular system) are formed in the rib zone. Stem cells located in the central zone divide and part of their daughter-cells are displaced into the peripheral and rib zones so as to replenish those regions; the other daughter-cells stay in the central zone and maintain a stem cell pool for future growth. The size of the stem cell pool is controlled by an underlying organizing center, marked by the expression of the transcription factor WUSCHEL, and a regulatory loop involving WUSCHEL and the CLAVATA receptor kinase pathway signaling cascade. ([1,5–7], for detailed reviews on the SAM).
Organ initiation begins with the selection of a group of founder cells in the peripheral zone, followed by the outgrowth of the incipient primordium and then the morphogenesis into a differentiated organ. Studies on living meristems revealed that initial primordium outgrowth is associated with oriented cell divisions; cell proliferation and cell expansion increase once the primordium outgrowth has started [4]. Organ boundaries with the SAM are marked by reduced cell expansion and division [4,8]. These patterns of cellular behavior are correlated with patterns of gene expression. In the organ founder cells, the KNOX meristematic genes, in particular SHOOTMERISTEMLESS (STM), are down-regulated [9,10]; these founder cells then start to express the ANT and the LFY transcription factors respectively associated with organ outgrowth and identity [11,12]. In the same time, the three CUC transcription factors are expressed in the boundary [13–15]. (for reviews, see [16] and [17,18] in this issue).
Lateral organs are arranged in regular patterns around the stem, a phenomenon called phyllotaxis from the Greek words “phyllo”, leaf and “taxis”, order. Various phyllotactic patterns are encountered in nature, namely alternate, opposite and spiral. Spiralled phyllotaxis, the most widespread one, follows a mathematical rule: consecutive lateral organs are formed with an angle close to 137.5°, the golden angle defined by the Fibonacci numbers [19]. At the Arabidopsis SAM, the first four leaves are formed as opposite pairs and then leaves and flowers follow such a spiraled phyllotaxis (Fig. 1).
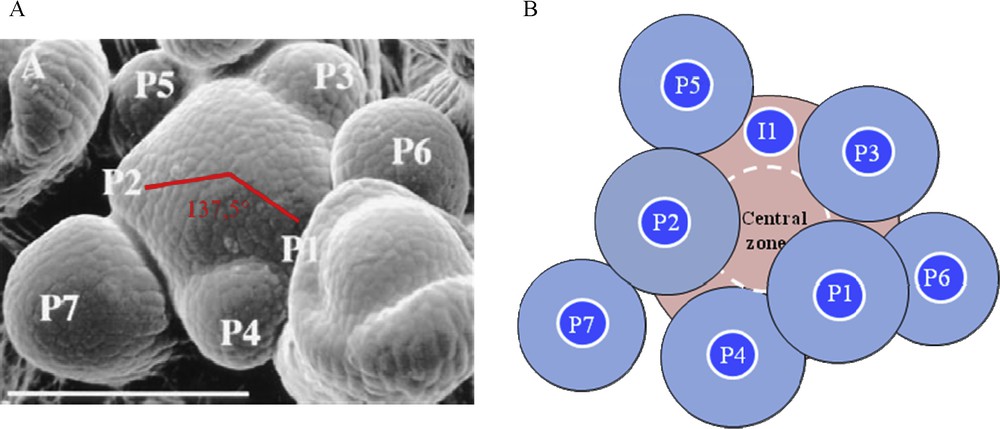
Phyllotaxis at the shoot apical meristem. A. Scanning electron microscopy of a young Arabidopsis inflorescence meristem. The meristem is the central dome. P indicates a floral meristem primordium, P1 being the youngest and P7 the oldest. Two consecutive primordia are separated by an angle close to 137.5°. Bar = 100 μm. From [3]. B. The inhibitory field theory of phyllotaxis. Shoot apical meristem is depicted in pink and organ primordia (P) in blue. Each preexisting primordium and the meristem central zone are surrounded by a hypothetical inhibitory field (light zone around primordia, dashed white line around the central zone). The future organ, called initium (I1), can only develop in the region without inhibitor.
What is the mechanism behind this regular spacing between primordia? Historical surgical experiments, consisting in removing an existing primordium, demonstrated that existing primordia determine future sites of organ initiation in adjacent regions of the SAM (for a review, see [20]). These results and others support a lateral inhibition model where developing organs and the centre of the meristem produce an inhibitory signal that acts locally to prevent organ formation. Due to the growth, existing organs move away from the meristem; new primordia can develop, far from the repelling effect (Fig. 1). This inhibitory field theory of phyllotaxis was postulated 80 years ago but the nature of the signal or signals involved remained unknown until recently. In this short review, we focus on the function of a very important signal, the plant hormone auxin (for detailed reviews on phyllotaxis [21–24]).
3 Auxin synthesis and transport
The major form of auxin identified in plants is IAA. Meristems, young primordia, vascular tissues and reproductive organs produce IAA [25]. IAA is then transported in the plant by two pathways: a passive one, in the phloem with the sap flow, and an active and directional one. This second process is called polar auxin transport; in stems, it occurs from the shoot apex towards the base of the plant.
The chemiosmotic hypothesis, proposed by Rubery and Sheldrake in 1974, provides an explanation for the molecular basis of polar auxin transport. Following the pH, IAA is present in its protonated form IAAH or in its anionic form IAA−. The pKa of this weak acid is 4.7. The pH in the cell wall is around 5.5; about 15% of IAA is in its IAAH form, an electrically neutral molecule which can easily diffuse into the cell through the membrane. In the cytoplasm the pH is around 7: IAAH totally dissociates into IAA−, a charged molecule which cannot pass through the membrane. As a result IAA− needs active efflux carriers to flow from cell to cell. It was proposed that the asymmetric membranous localization of these carriers would generate the polarity of auxin transport. The identification of polar auxin transport inhibitors such as the naphthylpthalamic acid (NPA) helped to confirm this hypothesis [26]. The observation of a saturable component for auxin uptake [27,28] and the identification of auxin influx inhibitors such as 1-naphthoxyacetic acid (1-NOA) [29] suggested that auxin influx carriers are also involved in polar auxin transport (Fig. 2); for reviews: [30–32].
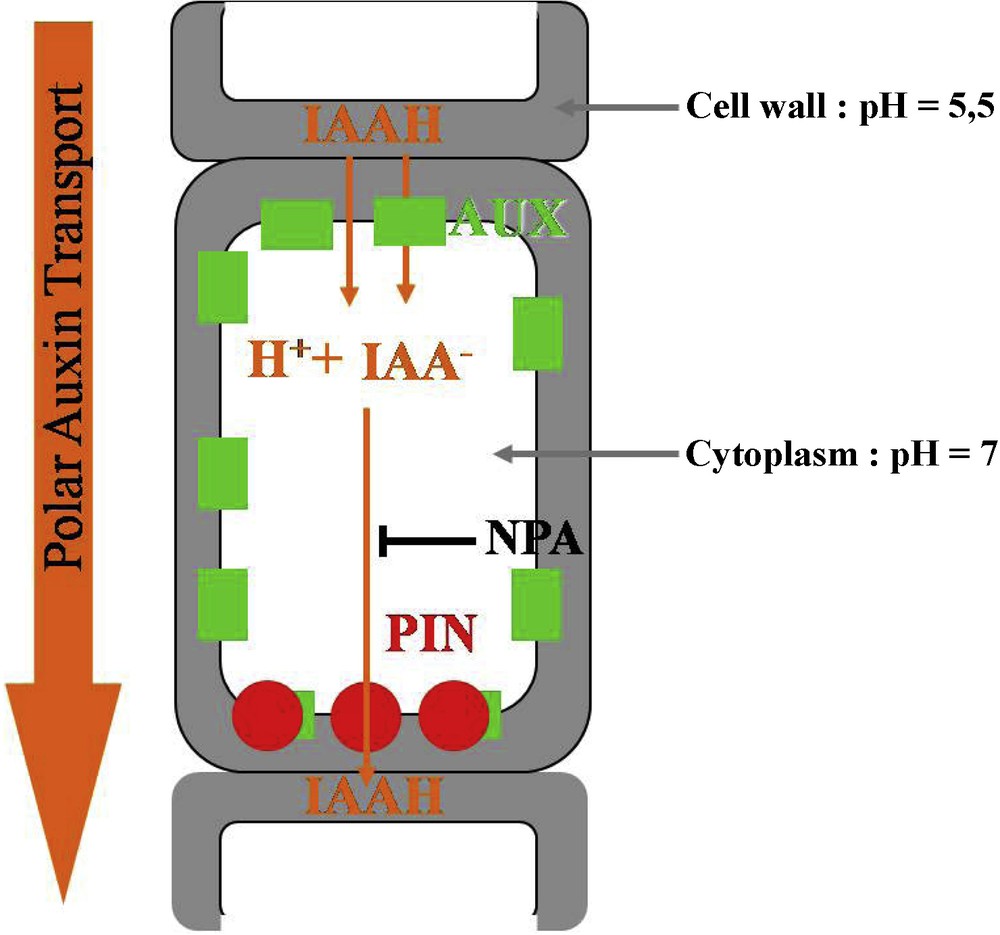
Polar auxin transport at the cellular level. IAAH enters the cell by diffusion or via the influx carrier AUX1. In the cytoplasm, IAAH tends to dissociate into IAA− and H+. IAA− can only exit the cell via the efflux carrier PIN, which has a polar localization. Polar auxin transport direction is controlled by PIN localization. NPA is an inhibitor of auxin efflux.
Molecular and genetic approaches subsequently permitted to identify and characterize the auxin influx and efflux carriers. Influx is under the control of the AUX1 protein, composed of 485 amino acids and 11 transmembrane domains [33]. The gene is expressed in the root and SAMs [34]; its expression in a heterologous system (Xenopus oocytes) leads to a saturable influx of auxin [35]. The lack of shoot phenotype in the aux1 mutant suggests that redundant factors could also be involved, probably LAX1, LAX2, LAX3 (Like AUX1) ([34]; for a review, see [27]).
The Arabidopsis pin-formed1 mutant (pin1), named in reference to its knitting-needle like inflorescence stem, shows a strongly decreased polar auxin transport. The culture of wild-type plants on the auxin efflux inhibitor NPA provokes the same phenotype [36]. The PIN1 protein, composed of 622 amino acids with eight to 12 putative transmembrane domains, shows similarities with certain bacterian and eucaryotes carrier proteins [37]. PIN1 is localized in a polarized way at the basal end of cells in stem tissues, as predicted for the efflux carriers by the chemiosmotic hypothesis [38]. PIN expression in yeast and mammalian cells induces an auxin efflux [39]. Genetic studies then demonstrated that a majority of the eight Arabidopsis genes (PIN1-PIN8) are auxin efflux carriers but differ in their expressions patterns [30,40,41]. Last, it has been directly demonstrated that PIN polarity directly determines the auxin flow [42].
Polar auxin transport is a highly regulated mechanism, although the molecular basis of PIN localization within the cell remains poorly understood. One of the most characterized regulators is the PINOID (PID) serine–threonine protein kinase, identified on the basis of the pin-like phenotype of the Arabidopsis pid mutants [43–45]. The analysis of PIN localization in the presence or absence of PID provided insight in its role. At the shoot apex, PID loss of function leads to an apical-to-basal shift in PIN1 polarity [46]. These results indicate that PID regulates polar auxin transport via a direct control of PIN localization. More recently it was shown that this involves the direct phosphorylation of PIN itself by PID [47]; for detailed reviews on polar auxin transport [31,32,48].
As briefly described above, the identification of the auxin efflux carrier PIN1 points to a role for auxin in organogenesis at the SAM. PIN1 gene expression is upregulated in the peripheral zone of the SAM at very early stages of organogenesis, even before the incipient primordium outgrowth has started: so far this is one of the earliest markers for organ formation [12,49].
4 Auxin and phyllotaxis
Inhibition of polar auxin transport, either by NPA or by mutations in PIN1 or PID, stops organ initiation at the flanks of the SAM but does not affect its other functions: it results in the formation of a naked vegetative or inflorescence stem [36,43,49,50] (Fig. 3). Exogenous microapplication of IAA to the apex of these naked stems restores organ initiation, showing that auxin is necessary and sufficient for inducing organogenesis. Another striking result is that organ initiation always takes place at the meristem flank, whether the IAA is applied to the peripheral zone or on the meristem summit [50] (Fig. 3). This suggests that the cells at the flank of the peripheral zone are competent to react to auxin, whereas cells at the central zone on top of the meristematic dome are not. Moreover, in the pin1 mutant, the two early markers of organ initiation LFY and ANT and the boundary marker CUC2 are co-expressed in the whole meristem periphery, suggesting that these competent cells have a hybrid organ/boundary identity. It is subsequently the amount of auxin applied that will decide whether or not a cell adopts organ or boundary identity [49]. If we interpret these results in the context of a wild type, a picture emerges, where local auxin distribution set up by polar auxin transport at the meristem periphery controls organ positioning and separation. In the next paragraphs we will further discuss what controls auxin distribution at meristem flanks.
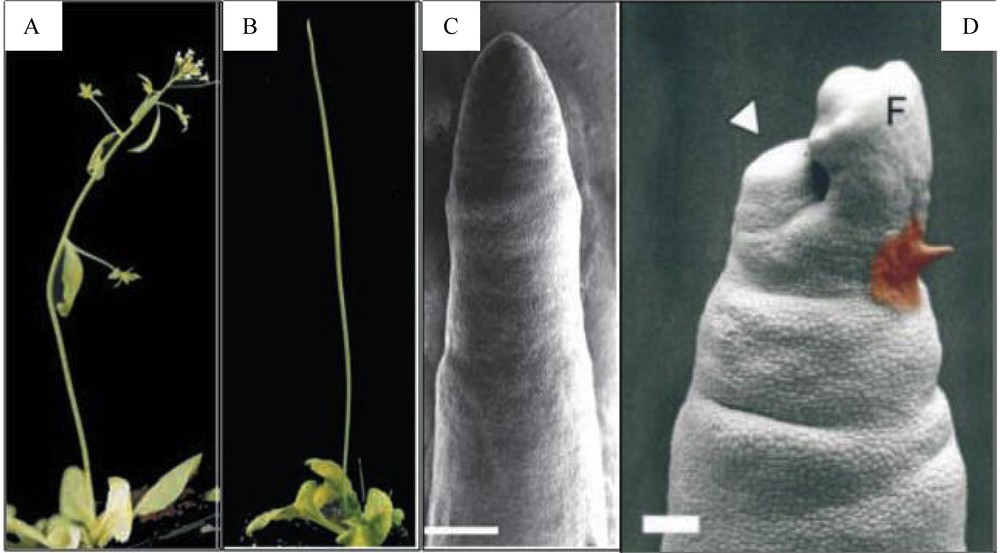
Importance of polar auxin transport in the shoot apical meristem for organogenesis. A. Arabidopsis wilt-type plant. B. Arabidopsis pin1 mutant; the stem has no cauline leaf, lateral branche and flower. C. Scanning electron micrograph of a pin1 inflorescence apex. The meristem is completely naked compared with a wild type SAM (see Fig. 1 A). Bar = 200 μm. D. Microapplication of IAA (1 mM, paste on right) at the flank of the SAM induces the initiation of a flower (result 4 days after treatment). Bar = 100 μm. A to C: from [49]. D: from [50].
Application of synthetic auxins as 2,4-D (2,4-dichlorophenoxyacetic acid) and NAA (naphthalene-1-acetic acid) at naked meristem flanks also provokes organ initiation but the primordia are ring-shaped [51]. These synthetic auxins have different properties regarding the polar auxin transport machinery: NAA does not need the influx carrier to enter into cells and 2,4-D does not use the efflux carrier [52]. The severely compromised localization and delimitation of primordia induced by those synthetic auxins confirm the importance of auxin local distribution for phyllotaxis and reveal the active implication of influx and efflux carriers in setting up local auxin gradients at the meristem periphery.
Powerful microscopic tools permit to visualize precise PIN1 localization in the meristem, either by immunolocalization with a PIN1 antibody or with the pPIN1::PIN1-GFP reporter gene. PIN1 is located at anticlinal sides of L1 cells and is also expressed in L2 and vascular tissues [53]. At presumptive sites of organ initiation, PIN1 is directed towards the initium center where it is strongly expressed [12] (Fig. 4). At the same time, DR5::GFP, an auxin-responsive reporter gene, is also strongly expressed, suggesting that auxin accumulates at these sites. This DR5::GFP expression pattern is abolished when auxin transport is inhibited by NPA treatment [12,54]. The complex patterns of PIN localization at the meristem were interpreted using computer simulations which confirmed earlier observations: PIN1 directs auxin to the sites of organ initiation [55]. Together, the observations suggest that auxin accumulation generated by PIN1 concentric distribution around incipient primordia is at the basis of organ initiation. Monitoring of PIN1 localization and DR5::GFP expression in living meristems reveals stereotypical PIN1 polarity changes correlated with auxin distribution changes and with the different stages of primordium development [12]. These results further indicate that PIN1 polarity, directing auxin accumulation and depletion in the meristem, controls phyllotaxis. Thus, an auxin maximum in the meristem periphery would provide the instructive signal for primordium initiation. Auxin would then be drained away from the surrounding cells by PIN1 via the young vascular tissues inside the primordium. As a result of this auxin depletion the neighboring L1 cells would not be able to initiate a new primordium at proximity. Instead of both an inductive and inhibitory signal as proposed by the inhibitory field theory, phyllotaxis would thus be controlled by a single positive signal, auxin. In this scenario, the postulated inhibitory fields around the primordia arise from draining away a positive signal (Fig. 5).
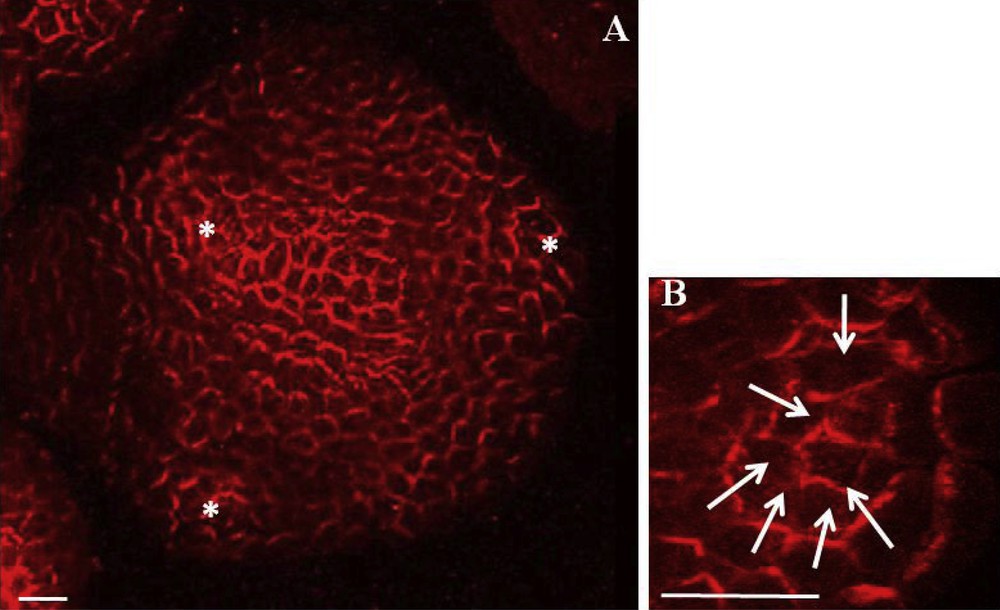
PIN1 localization in Arabidopsis shoot apical meristem. A. PIN1 immunolabeling on a meristem cross section showing L1. Asterisks indicate young primordia. PIN1 is localized on cell membranes and is mostly polarized. Bar = 20 μm. B. Detail of PIN1 immunolobaling showing PIN1 concentric orientation towards the centre of a future organ. White arrows indicate presumed local auxin fluxes. Bar = 10 μm. From [55].
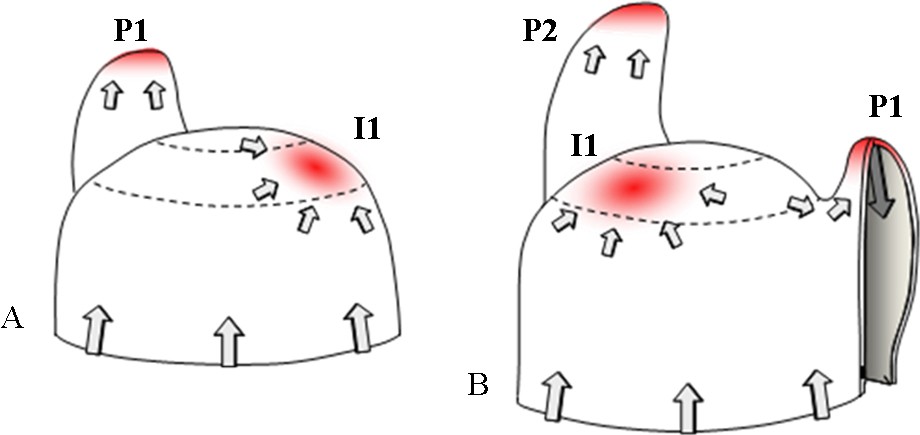
Model of the regulation of phyllotaxis by polar auxin transport in the shoot apical meristem. A. PIN1 directs auxin fluxes (grey arrows). Auxin accumulates (red) in the peripheral zone at the presumptive initiation site (I1, initium). B. PIN1 expression in vascular tissues inside the primordium evacuates auxin into inner layers, leading to an auxin minimum around P1. Another auxin maximum appears in the peripheral zone, determining the future organ initiation site following phyllotaxis. From [57].
A major question concerns the mechanisms controlling the auxin fluxes within the meristem. Recent computer models, developed to simulate phyllotactic patterns, provided interesting insights. Two models proposed that cells check the auxin concentrations in their direct neighbourhood, and subsequently PIN1 gets polarized so as to pump auxin towards the highest auxin concentration. This simple positive feedback loop is sufficient to generate different phyllotactic patterns [56,57] (for a detailed review, see [58]). Other models have since then been proposed. Stoma et al. showed that a model where cells sense auxin fluxes rather than auxin concentrations can equally reproduce the observed phyllotactic patterns [59]. More recently, Bayer and Kuhlemeier showed that a hybrid model, where both flux based and auxin concentration based PIN localization could also produce realistic patterning [60]. With regard to the role of the AUX1 importer, it has been proposed these could play a more discrete but nonetheless crucial role in phyllotaxis, by stabilizing auxin maxima generated by PIN1 distribution. Indeed, interference with auxin influx prevents proper localization and delimitation of primordia [51,53,61]. The quadruple mutant aux1-lax1-lax2-lax3 presents an altered phyllotactic pattern and a diffuse auxin distribution in the meristem peripheral zone; PIN1 distribution is also disturbed [62]. These results reveal a role for influx carriers in L1 auxin distribution, in interaction with PIN1.
Even if some mechanisms are still not completely understood, it seems now clear that phyllotaxis is mainly controlled by auxin distribution in the peripheral zone of the meristem. Polar auxin transport, in particular the dynamic PIN1 localization, generates auxin gradients which are then interpreted by meristematic cells in terms of differential growth and cell differentiation, leading to organ outgrowth and set up of boundaries.
Other factors, such as the hormones cytokinin and gibberellins, and mechanical constraints also influence phyllotaxis by modulating SAM size, tissue growth and cell differentiation [63].
5 Outlook: auxin and organogenesis at the root apical meristem
Auxin is not only crucial for shoot development but also in root development and patterning [64]. The organization of the root stem cell niche organization is different from the one in the shoot. The stem cells, called initials, surround an organizing center, called the quiescent center, which positions and maintains the stem cell niche. Stem cells asymmetrically divide and give rise to the various root tissues. In the embryo, specification of the basal root pole depends on the auxin gradient established by PIN1 and PIN7 [40]. During and after embryogenesis, PIN protein activity in the root leads to auxin accumulation at the root tip, which promotes the expression of the PLETHORA (PLT) transcription factors, major determinants for quiescent centre specification [65,66]. Auxin thus contributes to root stem cell niche specification and positioning (for reviews, see [67–69]).
The positioning of a new root does not follow a regular pattern as phyllotaxis. Lateral roots emerge in the differentiation zone from the pericycle, an internal cell layer. Pericycle cells locally re-initiate cell division and form root primordia with new stem cell niches, expressing PLT genes. Lateral root initiation depends on local auxin accumulation and detection in the pericycle [54]. Periodic fluctuations in auxin distribution and signaling along the root seem to regulate lateral root positioning [70]. ([1], for a review).
To conclude, it is worth noticing that local auxin gradients established by the dynamic polar auxin transport, especially the PIN efflux carriers, are involved in formation of all plant organs, lateral roots, leaves, and flowers, during post-embryonic development [54]. Interesting breakthroughs will now come from understanding auxin perception and cell competence.
Acknowledgements
I am grateful to Dr Jan Traas for a critical reading of the manuscript.