1 State of the art
Palynology is commonly employed as a tool for Quaternary palaeoecology and palaeoclimate reconstruction [1–6]. However, the interpretation of pollen data is sometimes difficult, as reconstructions have to take into account differences in pollen production and dispersal among plant species [7–13], pollen preservation, and definition of pollen source areas [14]. In addition, pollen records vary with vegetation types [9,14–19], size of the depositional basin, and other characteristics of the study area [9,20,21].
In mountainous areas, an additional difficulty is linked to tree pollen blown uphill from low-elevation vegetation belts into the sub-alpine and alpine belts. Therefore pollen spectra of the latter belts are dominated by non-local arboreal pollen. This phenomenon has been first recorded in the European Alps [22–24] and has been also found later in other mountainous areas in Africa, South America [25], New Guinea [26], and New Zealand [27,28]. In mountainous areas, climate varies strongly with altitude, largely determining the zonation of vegetation, reflected in turn by the pollen record at different elevations. However, if relationships between vegetation belts and relative pollen percentages in spectra from high-elevation sites are affected by local vegetation cover, they also depend on local slopes, exposure to dominant winds, and aspect [24,29–31].
However, whilst difficulties in implementing pollen-based reconstructions of the vegetation and climate history from alpine and subalpine sites have long been known [23,32–34], very few studies of modern pollen rain have been attempted in mountain environments of southern European countries [35–37]. Consequently, whilst pollen-based reconstructions of Holocene climate yield good results when applied to pollen sequences from low to mid elevation sites, the application of current methods to high-altitude pollen sequences (above 1000 m a.s.l.) often gives unreliable results [24,37–40]. Analyses of modern pollen rain are thus essential for the understanding of fossil pollen sequences in a particular region, and thus for pollen-based palaeoenvironment and palaeoclimate reconstructions. In this aim, moss polsters are commonly used as surface samples for local modern pollen deposition [41,42]. They are assumed to record an average of several years of pollen deposition [9,43] and to be a good analogue of fossil pollen assemblages.
Based on the analysis of modern surface pollen spectra from two areas sampled on the two sides (French and Italian) of the north-western Alps, this work aims to provide an understanding of elevation-induced variations of pollen spectra composition to estimate the real potential of: (1) quantifying and reproducing the relationship between pollen-assemblage changes and the altitudinal climate gradient in different regions of the Alpine area; and (2) estimating the effect of local parameters on the relationships linking pollen percentages variations, elevation and climate.
2 Study area and material
Twenty-three of the 37 modern surface pollen samples from the Aosta Valley (AO) were collected in forest openings below the treeline, and in open landscapes above the treeline, along an altitudinal transect from 1177 to 2680 m a.s.l. (a.s.l. is subsequently omitted in the text) in the upper part of the Valsavarenche Valley, in the southern area of the Gran Paradiso National Park (south-western Aosta Valley, Italy) (Fig. 1). Species nomenclature follows Flora Europaea [44]; species names are followed by the author when they are cited for the first time. The transect recorded various vegetation formations, developed along the altitudinal gradient (see following paragraph). Relict broad-leaved formations occurred in the lowland (below 1500 m), with conifers over 1300 m; spruce (Picea abies (L.) Karsten)-dominated formations with larch (Larix decidua Miller) developed at 1500–1800 m. Larch-dominated woods were found between 1800 and 2150 m, with shrubs such as Rhododendron ferrugineum L., Vaccinium myrtillus L., Juniperus communis L., and herbs forming the understory above 2000 m. The larches gave place over 2300 m to shrub-dominated communities with Salix hastata L. and typical alpine pasture taxa like Trifolium alpinum L., Carex curvula All., Nardus stricta L. and Leontodon pyrenaicus Gouan ssp. helveticus (Mérat) Finch. The upper limit for isolated trees was around 2415 m, and above this alpine pastures became dominant. Above 2500 m plant communities were dominated by Poaceae and Cyperaceae and characterised by typical alpine herbaceous taxa (Silene acaulis (L.) Jacq., Trifolium alpinum, Myosotis alpestris gr., Alchemilla vulgaris gr., Gentiana verna L., Leucanthemopsis alpina (L.) Heywood, Viola tricolor L., Sagina sp., Saxifraga sp., Rumex alpinus L., Trifolium pratense L. ssp. frigidum Gaudin, Artemisia glacialis L.). 14 supplementary modern moss samples from various peat bogs in the eastern region of the Aosta Valley were added from the work by Brugiapaglia [45]. These samples came from locations at different elevations and in different vegetation communities: broad-leaved formations in the lowlands (below 1000 m), mixed deciduous forest with conifers (below 1500 m), conifer woods with P. abies and L. decidua (1800–2300 m), alpine pastures with rare larch trees (over 2300 m). All the localities sampled in the Aosta Valley are shown in Fig. 1.
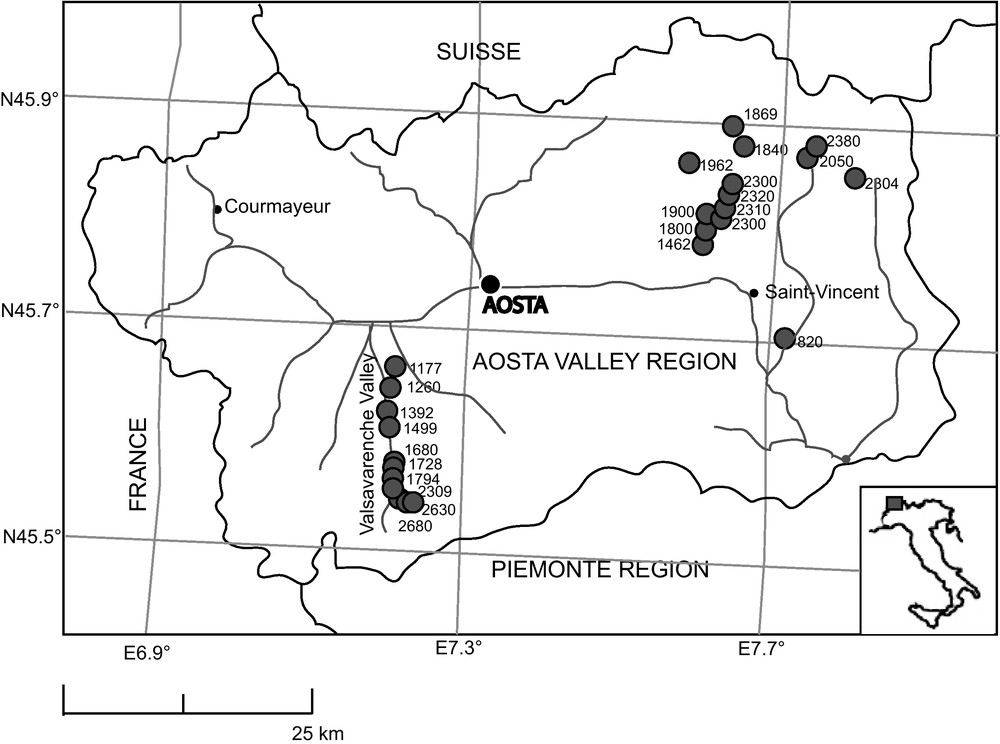
Location of the sites sampled for modern pollen rain in the Aosta Valley area (including the Valsavarenche; Italy) with indication of the altitudes (in m a.s.l.) of the sites studied.
The 40 modern pollen samples from the Taillefer Massif (TA) (Fig. 2) were collected along an altitudinal transect from 350 to 2600 m across the following phytocenoses: broad-leaved formations with Quercus, Carpinus, Tilia, Castanea (below 1000 m), Fagus woods with Acer, Fraxinus, Corylus (below 1300 m), Picea woods (below 1800 m), open conifer formations with shrubs in the understory (Picea abies, Pinus cembra, Rhododendron ferrugineum, Vaccinium myrtillus) and alder formations (Alnus viridis (Chaix) DC., Rumex arifolius All., Geranium sylvaticum L.) (1800–2000 m), alpine pastures with shrubs (Nardus stricta, Luzula sp., Rhododendron ferrugineum, Vaccinium myrtillus) (2000–2350 m), alpine discontinuous communities (Minuartia sp., Sempervivum arachnoideum L., Silene acaulis) (2350–2600 m). The collection procedure for the palynological material followed Heim [9].

Location of the sites sampled on the Taillefer Massif (France) with indication of the altitudes (in m a.s.l.) of the sites studied.
The Taillefer region is located between two extreme climatic regions: in the north-west, rainfall values are higher (1600 mm/year), while the south-west is more arid (800 mm/year). The dominant winds are from NW to SSE. This high precipitation justifies the present-day occurrence of large broad-leaved formations with Castanea sativa Miller and Carpinus betulus L. in the hills, Fagus sylvatica L. woods in the montane belt, and Picea abies woods with Abies alba Miller formations in the lower subalpine belt. Shrubs including Rhododendron ferrugineum, Juniperus communis L. ssp. nana Syme, Vaccinium myrtillus, Vaccinium uliginosum L. and Vaccinium vitis-idaea L., are found in the upper subalpine belt. A complete description of the vegetation surrounding the sampled points is given by Brugiapaglia et al. [24].
Vegetational differences between the two study areas are mainly determined by the climate, which is typical of inner alpine zones in the Aosta Valley, with yearly rainfall values close to 600–800 mm in the central parts and up to 1000–1200 in other parts, near the borders with France and Switzerland; this accounts for the scarcity of broadleaf forests and the occurrence of Larix decidua (planted at low elevations) and of Pinus sylvestris L. woods replacing (because of lower precipitation) the beech and mixed broadleaf forests, which are dominant in adjacent valleys (which have a different orientation and position within the Alpine chain, resulting in higher precipitation) [46]. In the eastern region of the Aosta Valley, Fagus sylvatica and Abies alba are almost totally absent due to dry conditions, while Larix decidua and Picea abies formations are widespread [47].
Although human activity has favored the spread of pioneer taxa throughout the Alps by extensive deforestation, their development is in any case subject to climate and to interspecific competition: the sum of these factors results in the development of larch and pine in areas where climate conditions are favorable, while more demanding taxa (e.g. P. abies or Abies alba) do not find optimum conditions [46]. The influence of climate and soil is still present despite human activity.
3 Methods
Moss samples were collected in the various vegetation formations developed at different elevations in the Alps.
The coordinates of each sample point were established by GPS, the altitude was then verified on maps. Pollen and spores were extracted by physical-chemical treatments from moss samples, including cold 36% HCl (24 h.), cold 70% HF (48 h.), 10% NaOH (10 min. at 95 °C) and acetolysis during 8 min. at 90 °C, on the organic and inorganic material, and sieving at 200 μm. At least 400 terrestrial pollen grains were counted per sample under a light microscope. Pollen grain identification was based on photographs by Reille [48–50] and by comparison with specimens from the Laboratoire Chrono-Environnement (French CNRS and Franche-Comté University, France) and the IMEP (French CNRS and Aix-Marseille 3 University, France) reference collections. Pollen diagrams (Fig. 3) were drawn using the GpalWin program [51]; percentages were calculated on the basis of total arboreal and non-arboreal pollen grains. Based on pollen morphology within the Cyperaceae, it is not possible to distinguish taxa that dominate alpine pastures plant communities (e.g. Carex curvula) from taxa typically developing in peat bogs (e.g. Carex nigra (L.) Reichard). Therefore, despite its importance in some modern pollen spectra from alpine pastures communities, we decided to exclude the Cyperaceae group from the total pollen sum in modern pollen spectra, in parallel with the exclusion of this group when analysing fossil pollen samples for palaeoenvironment and palaeoclimate reconstruction [52]. Pollen frequencies lower than 1% are represented by points.

Comparison of pollen assemblage variations with elevation in the two study areas: only the most represented taxa are shown.
Correspondence Analysis (CA) and Cluster Analysis were performed on pollen data to underline statistical similarities and differences between pollen assemblages within comparable vegetation belts in the two areas. These numerical classifications were applied to the data of the two areas (AO and TA) to quantitatively group similar modern surface pollen spectra with respect to their composition and to the proportions of the various taxa in pollen floras. To avoid overestimation of high-frequency taxa, the pollen data were ln (x + 1)-transformed before CA processing.
3.1 Elevation calculation
Based on all the taxa present in the modern pollen assemblages and considering the different alpine environments of the Aosta Valley and the Taillefer, a transfer function from pollen percentages to elevation was calculated using forward multiple linear regression.
3.2 Calculation of temperature values
The lapse-rate induced decrease in temperature was calculated from observed climate data (from the New LocClim 1.06 [53] database) at stations at various elevations on the western alpine chain. The average altitudinal decrease in temperatures obtained per 100 m (mean temperature of January: TJan: 0.46 °C, mean temperature of July: TJul: 0.67 °C, mean annual temperature: Tann: 0.57 °C) is consistent with climate gradient observations by Ozenda [54]. Temperature values for the sites at lower elevation were interpolated by the New LocClim 1.06 program for local climate estimation [53]. Temperatures for each sampled point were then calculated from its elevation applying the vertical temperature gradient described above.
Three transfer functions were calculated by different methods on the basis of the Aosta Valley (AO) (including the Valsavarenche Valley) and Taillefer (TA) pollen assemblages to predict Tann: transfer function f1 with a forward step linear multiple regression, transfer function f2 with backward step linear multiple regression, and transfer function f3 as a backward multiple linear regression with a tolerance level of 0.001 (where the tolerance of a variable is defined as 1 minus the squared multiple correlation of this variable with all other independent variables in the regression equation).
4 Results
4.1 Pollen diagrams
The pollen percentages for various taxa from the Aosta Valley were plotted against the elevation of the sampled sites; the diagram is shown in Fig. 3, compared to the pollen diagram obtained from the Taillefer Massif along an altitudinal scale gradient. Only pollen taxa occurring in most of samples are shown. The pollen taxa are ordered, when possible, following their altitudinal distribution.
Based on a classical zonation, it is possible to recognize five altitudinal pollen zones showing a common background in the two diagrams:
AO1, TA1 (0–1500 m): This zone was characterised by important percentages (5–30%) of broad-leaved taxa (Fraxinus, Corylus, Castanea, Betula) and herbs related to anthropogenic low elevation meadows (Poaceae, Rumex). Fagus was well represented in the Taillefer pollen data, while it was rare in those of the Aosta Valley
AO2, TA2 (1500–1800 m): Picea dominated this zone in the two diagrams, showing maximum percentages between 30 and 70% at around 1700 m. Low percentages of Larix (6% on average) characterised the Aosta Valley sequence, while this taxon was absent in the Taillefer. A. viridis, Plantago and Apiaceae appear from 1500 m in the two diagrams
AO3, TA3 (1800–2150 m): The increase of A. viridis and Poaceae was common for the two areas at these elevations. Larix and Pinus characterised the AO3 pollen zone, while the absence of Larix on the Taillefer and high percentages of Poaceae were notable in the TA3 zone
AO4, TA4 (2150-2500 m): The increase of herbaceous taxa was more evident in zone AO4 than in zone TA4, where an increase of Pinus pollen was noted. Herbs, however, dominated the pollen assemblages at this elevation in the two areas
AO5, TA5 (2500–2680 m): These zones showed a decrease of Poaceae and were characterised by important percentages up to 40% of Caryophyllaceae
4.2 Floristic composition (using Cluster Analysis)
Fig. 4 shows the results of cluster analysis on the whole pollen samples from the Taillefer Massif and the Aosta Valley. Pollen samples from alpine pastures are often gathered with samples from meadows and open conifer formations with shrubs, while, except very few samples (TA850, TA1150, AO1260, AO1391, AO1885), pollen spectra from the following vegetation units are separated into distinct groups: anthropogenic lowland landscapes with deciduous taxa, spruce-forests, alpine pastures with shrubs, higher lands that are dominated by Caryophyllaceae.
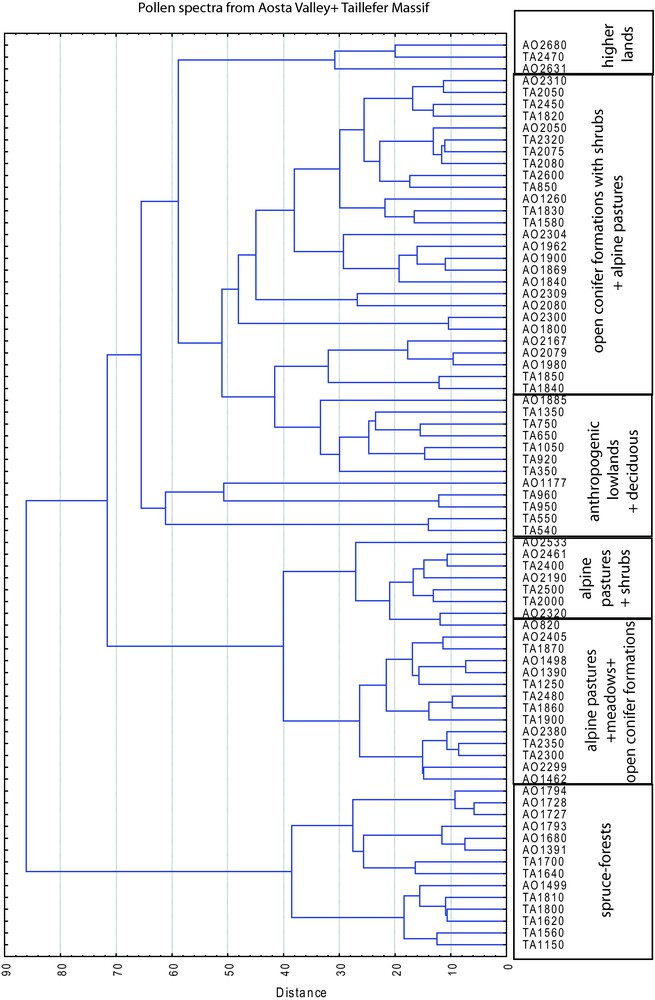
Cluster analysis performed on the whole pollen dataset, showing a consistence with the pollen diagram altitudinal zonation, except for samples from low altitude meadows, several alpine pastures and open conifer formations that are often gathered together.
4.3 Floristic composition (using Correspondence Analysis)
Fig. 5 shows the results of the CA where the first two axes yielded the highest amount of explained variance (first axis ∼15.8%, second axis 12.4% related to 77 assemblages and 88 taxa, or if only taxa that contributed with more than 5% in sum across all 77 assemblages are considered, then first axis ∼16.4%, second axis 15% for 37 taxa). Fig. 4 shows that the pollen data from the two areas AO and TA fell into two groups due to differences in their floristic composition. However, a common trend due to similar variations in pollen assemblages that can be related to elevation is suggested.

Dispersion of samples from CA performed on the whole pollen dataset (88 taxa in 77 samples, Cyperaceae excluded). The groups of points show a trend (arrow) following the elevation of the sites sampled.
Floristic composition-elevation: In order to analyse the relationship between elevation of the samples and their community composition, the elevation of each sample was regressed against its CA axes scores (Fig. 6). Whilst a good relationship between pollen assemblages and elevation is found for the two areas, results show a closer relationship with elevation for the Taillefer pollen data than for the Aosta Valley.

Results of CA analysis first (a) and second (b) axes values plotted against elevation.
Pollen-elevation (using Multiple Linear Regression): For the calculation of a multiple linear regression between altitude and modern pollen spectra from AO and TA, only common taxa have been used with each an occurrence higher than 5% in sum across the 77 assemblages, resulting in a total number of 47 taxa used. The result (Fig. 7) shows a highly significant correlation of R2 > 0.9 between predicted and observed values, with a standard deviation of 145 m to the true elevation. It can be observed, however, that Taillefer pollen assemblages from elevations below 1000 m a.s.l. show the highest deviations from the regression line. This is highlighted in Fig. 7 by the regression line and its coefficient of determination solely calculated from these assemblages.

Coefficients of determination (R2) calculated on observed and pollen-inferred altitudes for all sites: results from standard multiple linear regression.
Pollen-temperature: The application of transfer functions f1, f2 and f3 established on the basis of the (calibration data set of) AO pollen data, on the TA (testing) data set yields very different results for the three different transfer functions with f1 being based on 24 taxa and having a R2 of 0.13, f2 (27 taxa-based and R2 of 0.62) and f3 (24 taxa-based and R2 of 0.71). As an example, the results of the application of f2 to the Taillefer Massif pollen data are shown in Fig. 8, showing that pollen-inferred temperature values based on this transfer function are consistent with the observed ones for samples collected over 1000 m a.s.l.

Pollen-inferred annual temperature based on transfer function f2, compared to actual temperature (calculated as explained in the method section) on the Taillefer Massif sites.
5 Discussion
5.1 Similarities and differences in pollen assemblages composition for the two areas
Figs. 3 and 4 show the good correlation between the altitudinal pollen zones deduced from the modern surface pollen assemblages of the Taillefer Massif and the Aosta Valley, while graphics in Figs. 5 and 6 show differences in the composition of pollen assemblages from these two areas, yielding two well-separated groups of points. This is linked to the different weight that cluster and correspondence analyses give to the qualitative and quantitative composition of pollen spectra from the two areas: CA underlines more the differences in pollen flora composition, while cluster is more influenced by pollen percentages. Considering the variations of pollen percentages along the altitudinal gradient, it appears that some pollen taxa are present at most elevations, but are only abundant within limited elevation ranges. Examples of pollen types with rather narrow representation maxima can be found for most elevation ranges (e.g. Picea around 1750 m, Caryophyllaceae around 2600 m). The results from cluster better underline similarities between samples coming from the same vegetation belts as reflected by pollen spectra, while CA shows that pollen spectra vary independently in the two areas and that these variations are induced by the altitudinal gradient. Besides, cluster does not put in evidence the difference between lowland spectra that are often dominated by anthropogenic meadows (Poaceae dominant) and alpine pastures (Poaceae also dominant), or between these grasslands and open conifer formations (Pinus represented by high pollen percentages in the two cases). Generally, CA considers this difference, as the classification of samples in groups of points is not only led by dominant taxa but also by the qualitative composition of the assemblages, and then takes into account taxa that are quantitatively under-represented in pollen spectra, but typical of a particular elevation range. Some spectra from deciduous formations were also gathered by the cluster with spruce-forests or other conifer-dominated formations ones, and vice-versa, probably due to anthropogenic disturbance or the effect of local factors on pollen/vegetation relationship.
The results also indicate that the floristical differences between the two areas and maybe also the width of the area chosen for sampling (a massif for the Taillefer pollen data, an area enlarged from a valley to a region for the Aosta ones) might have an influence on the reproducibility of the relationship between the composition of pollen assemblages and the elevation of sites. The lack of samples below 800 m a.s.l. from the Aosta Valley, might also influence the R2 score. Fig. 5 shows the results of CA applied to the whole pollen data set: pollen samples from the two areas are separated in two different groups of points, whose trend suggests a considerable direct relationship with elevation. The regression of CA axis 1 against elevation (Fig. 6) shows a strong relationship between the change in pollen assemblages’ composition and the altitudinal gradient. The relationship is stronger for the Taillefer Massif data than for the Aosta Valley data. This might be linked to the fact that the sampled area was wider for the Aosta Valley region providing a higher number of surface pollen assemblages from different vegetation communities than for the Taillefer, but an influence of the stronger anthropogenic impact that affected the lowland of the Aosta Valley region can also be seen. In the Aosta Valley region, the natural broad-leaved forest that represents the potential vegetation in the lowlands has almost completely been replaced by anthropogenic meadows [47] and by planted L. decidua woods. Although we avoided sampling in clearly anthropogenically altered areas, the presence of meadows is anyway evident in high Poaceae percentages in pollen assemblages from the montane belt (Fig. 3), due to wind-driven pollen transport.
5.2 Lapse-rates induced variations in pollen assemblages
A good correlation of the main vegetation formations represented by pollen zones in the two areas of the Aosta Valley and the Taillefer Massif is clearly shown on Figs. 3 and 4. Figs. 5 and 6 show that a strong relationship exists between altitude and pollen assemblages that can be used as a tool to reconstruct the elevation of a site from its pollen rain composition (supported by Fig. 7), yielding good results (R2 > 0.93; standard deviation: 145 m). Based on these results, we can affirm that pollen assemblages from the same altitudinal range in the two study areas are characterized by a common background. The relationship between pollen assemblages and elevation seems not only due to the taxa that are dominant in each altitudinal zone (e.g. Picea in zones AO2 and TA2), but also to the presence of low percentages of companion species, whose presence or absence can be linked to the elevation. The validity of this last affirmation is shown in Figs. 3 and 4 by the presence of many common taxa in pollen zones corresponding to the same altitudinal ranges in the two areas (e.g. Alnus viridis, Plantago, Apiaceae in zones AO2 and TA2), and is also supported by the graphic in Fig. 7, showing that the absence, in one of the two areas, of one taxon that is well-represented in the second area (e.g. Larix present in AO2, absent in TA2) do not prevent to underline the strong relationship with altitude that pollen assemblages from the two areas show. This can be imputed to the actual vegetation zonation as well as similar wind-driven pollen transport mechanisms in the two areas.
5.3 Use of pollen rain for the reconstruction of mean annual temperature (Tann)
Since the temperature decrease with elevation is known to be linear [54] in the mountainous area of the Alps, an altitudinal zonation of the vegetation appears, which is reflected by pollen percentages variations with altitude. Related to this relationship, also a linear relationship may be supposed to exist between pollen assemblages and temperature. However, due to floristical differences between the two areas, the mean temperature inferred from pollen data from one region might not be in consistence with the actual temperatures recorded in the other one.
Three different transfer functions were elaborated following the procedures explained in the “Methods” section, based on the Aosta Valley pollen dataset exclusively. Their application to the Taillefer Massif pollen data yielded very different results for the three functions. The transfer function 1, calculated with forward step, yielded very bad results compared to the two other ones; transfer function 3 yields very reliable results, but it is based on a small number of taxons. We show the test of transfer function 2, as a good compromise between number of taxa and R2 value. Fig. 8 clearly shows that the reliability of the elaborated transfer functions is higher for samples over 1000–1500 m, that means at altitudes where difficulties in reconstructing temperature from pollen data are commonly underlined.
Climatic and floristic differences between the two areas exist, that may explain the different results when directly applying the transfer functions pollen/mean annual temperature elaborated on the first area to the second one. This might be due to the fact that mean annual temperature is not the only factor having an influence on the vegetation distribution: the ecological tolerance of various taxa is also limited by other factors such as precipitation and insolation, and the actual distribution of taxa in the two areas is probably due to the combination of them. Although a bias can be invoked, depending on the importance of human disturbance on the lowland, over 1500 m, altitude seems to become the dominant factor having an effect on the vegetation altitudinal distribution which is recorded by pollen spectra variations. A purer relationship between altitude and temperature decrease over 1000 m can also be found when observing modern climate data from meteorological stations; under 1000 m a.s.l., the effect of lapse rates is not so clearly underlined [55].
The method seems then to give a reliable reconstruction of the temperature gradient based on pollen data. However, differences in the reliability of the estimations obtained when slightly changing the floristical composition of pollen samples or the elaboration of the transfer functions suggest to be careful when applying them to fossil data, since important variations affected the vegetation composition during time, due to climate fluctuations and human disturbance.
More tests, taking into account the maximum of taxa, are needed to underline the weight that the various taxa can have on the elaboration of transfer functions towards temperature, and to obtain a method that might be applied to the various situations in the Alpine area during the Holocene period.
6 Conclusion
The results show that pollen assemblages, from two different regions located in the North-western Alps (the Aosta Valley and the Taillefer Massif), where the local vegetation distribution along the altitudinal climate gradient is similar, are similar enough to produce a parallel trend in the dispersion of points when analysed by CA. Among the multivariate analyses applied to pollen data, CA seems to be most adapted to characterise the altitude-induced floristical variations in pollen assemblages in each area, while Cluster Analysis well underlines the characterisation of the various belts and gathers spectra from the two areas based on their common background related to the altitudinal range to which they belong; at the same time Cluster does not always underline differences between lowland meadows, alpine pastures and open conifer formations, while this difference is better underlined by CA. The application of multiple linear regression to the whole pollen dataset shows that a linear relationship exists between pollen assemblages’ variations and the elevation of the study sites; the altitude of each site can be reconstructed from pollen data within an error of 145 m. Based on this relationship, three different transfer functions were elaborated from pollen spectra to annual temperature values; a good compromise for the application of the transfer function elaborated on one region (the Aosta Valley) to the other one (the Taillefer Massif) was obtained with a transfer function based on a linear multiple regression created with backward step. The results show a good reliability of the temperature estimated from pollen data; the comparison to the observed values shows that the reconstruction is more reliable over 1500 m a.s.l., probably due to the human disturbance on the lowland landscape as well as to the less important effect of the lapse rates-induced climate variations on the vegetation distribution and on pollen data. Since R2 > 0.6 when predicting temperature parameters from pollen, we might suppose that the remaining < 0.4 (thus < 40%) variance of explanation is due to other local factors such as geomorphology, phenomena like temperature inversions or the aspect of slopes, the direction of winds, but also to differences in the pollen flora from the two areas. The results obtained in this work show that the relationship between pollen assemblages, elevation and temperature is quantifiable and reproducible. However, the differences in the reliability of the various transfer functions due to little variations in the pollen flora or in methodology suggest that one should be careful in the application to fossil data. Our results underline, once more, that transfer functions for palaeoclimate reconstructions should be established on modern analogues from the same area than the fossil assemblages. More modern pollen data will be necessary to produce relationships reliable for different alpine regions.
Conflict of interest statement
Nothing declared.
Acknowledgements
This work has been carried out within the project “Relations between climate change, ancient and actual vegetation, on the basis of phenological and palynological data” financially supported by the Regione Piemonte (responsible R. Caramiello). Part of the samples collection has been financially supported by the French CNRS within the framework of the ECLIPSE Program (Past Environments and Climates).