1 Abbreviations
VGSC
Voltage-gated sodium channels
VDWVan der Waals
ECDExtracellular domain
CAMsCell adhesion molecules
ZDOCKMolecular docking software
3MJ7-AComplex of JAML and Coxsackie and Adenovirus receptor CAR, chain A; 1NEU-A, Myelin Membrane Adhesion Molecule P0, chain A
PDBProtein Data Bank
IDIdentity
BLASTBasic Local Alignment Search Tool
NCBINational Center for Biotechnology Information
GEFS+Generalized Epilepsy with Febrile Seizures Plus
2 Introduction
In vivo, most Na+ channel α subunits (Nav1.1–Nav1.9) are associated with one or more β subunits; nowadays, four distinct isoforms (β1∼β4) and two splice variants (β1A, β1B) have been discovered [1,2]. Structure/function studies have identified the P loop of domain IV of the Na+ channel α subunit as a likely interaction point for the extracellular domain of β1, accounting for the fine-tuning of Na+ channel activation and inactivation gating [3]. More recently, people began to focus on the studies of β subunits and their mutants, as experiments showed that many diseases were associated with β subunits changes, especially in the extracellular domain (ECD), which is in charge of the electrophysiological properties of the sodium channel and cell adhesion or migration [4].
As we all know, the β subunits share a common structure: a single membrane domain, an intracellular C-terminal domain, and a large extracellular N-terminal domain (Fig. 1). The ECD contains four potential N-glycosylation sites, which were modified with sialic acid; in this domain, they all have an immunoglobulin-like fold [5,6]. Among these subunits, β1 and β3 are non-covalently linked to VGSC α subunits, while β2 and β4 associate with disulfide connection. Particularly, β1 and β3 have compact relations; they are structurally similar, with 57% sequence identity and related to the same diseases, such as epilepsy and cardiac arrhythmia [4,7,8]. Recent studies reveal that the β1 and β3 subunits are always co-expressed in many tumour cells and involved in epilepsy, cardiac arrhythmia [6,9]. So, we speculate that there must be a special interaction mode between β1 and β3. To prove this inference, we have to know the crystal structure model of β1 and β3; however, there have been so far no published studies about 3-dimensional structure and interaction analysis between β1 and β3.
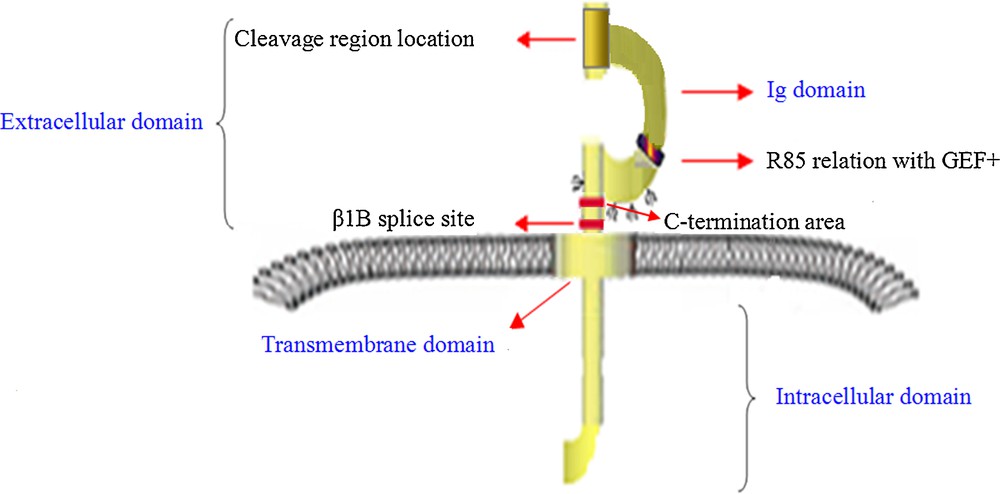
(Color online.) Basic functional architecture of β1 and with highly conservative structure domain like other β subunits.
Therefore, in the present study, we provide a hypothesis that β1 has certain relationship with β3 subunit, which is different from the results of McEwen et al., who studied the interaction between β subunits and other CAMs in glial cells [10]. Moreover, this paper screened out the homologous mode template of β1 and did the docking studies of β1 and β3 subunits, which provides a strong basis for the further research of β subunits on structure and functional drug targeting.
3 Materials and methods
3.1 Molecular modelling
The amino acid sequences of β1 and β3 were obtained from the database in the National Centre for Biotechnology information. The protein BLAST program against Protein Data Bank available at NCBI was used to select a template structure for homology modelling of β1 and β3. From the selected templates, the three-dimensional models of β1 and β3 were obtained by homology modelling using software package Discovery Studio 2.5. The obtained models were both validated using PROCHEK subsequently [11].
3.2 Dynamics and PROCHECK
In order to demonstrate the more reliability of the newly screened template, we had carried out the dynamic optimization of the β1 modelling structure. The force field was based on FF99SB and the time step was 2 fs. A constant pressure of 1 bar, independently in the z direction, was used with a coupling constant τp = 20 ps. Water, lipid, and protein were coupled in a temperature bath at 310 K, using a coupling constant τt = 0.1 ps. Finally, the stability and reliability of this system were demonstrated.
3.3 Protein–protein docking
To generate the docking model between β1 and β3, the ZDOCK program in the Discovery Studio 2.5, which requires minimal information about the binding sites, was used. Finally, ZDOCK used Pairwise Shape Complementarity, desolvation and electrostatic energy methods to evaluate the proteins interaction [12–14].
4 Results
4.1 Molecular modelling of β1 and β3
Molecular modelling of β1 was carried out based on the crystal structure of the complex of JAML and Coxsackie and Adenovirus receptor, CAR of Mus musculus (PDB ID: 3MJ7) chain A [15], which shares 22% sequence identity with β1 (29Glu to 152Arg), while the model of β3 was based on the crystal structure of myelin membrane adhesion molecule P0 (PDB ID: 1NEU) chain A [16], which shares 28% of sequence identity with β3 (25Val to 147Glu), (see Table 1). In addition, two crystal models were obtained by comparing screening, respectively potassium ion and calcium ion channel current β subunits.
Template screening statistical information of ion channel β1 subunit.
Channel category | PDB ID | Identity ratio | 3D structure |
Voltage-dependent calcium channel-beta subunit | |||
Beta subunit isoform 2aa | 1T0H | 12.9% | |
Beta subunit functional coreb | 1T3S | 12.3% | |
Beta-2 subunit in complex with the Cav1.21-lid linkerb | 4DEY | 12.2% | |
Beta2a subunit and a peptide of the alphalc subunita | 1T0 J | 11.3% | |
Beta4a-A domainc | 2D46 | 11.0% | |
Beta-2 subunit in complex with the Cav2.21-lid linkera | 4DEX | 10.9% | |
Beta3 subunita | 1VYU | 9.7% | |
BK Beta subunit Kcmnb 2c | 1JO6 | 9.6% | |
Beta3 subunit complex with aida | 1VYT | 4.8% | |
Voltage-dependent potassium channel-beta subunit | |||
Beta subunit (I211r) IN Complex with cortisonea | 3EB4 | 15.9% | |
Kv1.2 potassium channel-beta subunit complexa | 2A79 | 15.4% | |
Full-length shaker potassium channel Kv1.2a | 3LUT | 15.4% | |
Structure of the cytoplasmic beta subunit-tla | 1EXB | 15.4% | |
Beta subunit IN complex with cortisonea | 3EAU | 15.3% | |
Beta subunit (W121a) IN Complex with cortisonea | 3EB3 | 15.3% | |
Paddle chimera channel IN association with beta subunit § | 2R9R | 6.3% | |
Structure of a votage-dependent+ channel-beta subunita | 1QRQ | 4.6% | |
Ig superfamily | |||
Crystal structure of the complex of Jaml and CARd | 3MJ7 | 22.2% | |
Crystal structure of the extracellular domain from P0a | 1NEU | 28% |
a Rattus norvegicus.
b Oryctolagus cuniculus.
c Homo spaiens.
d Mus musculus.
Analysis of the Ramachandran plot showed that 73.3% and 80.6% of the residues were in the most favourable regions, with 21.6% and 14.8% in the additional allowed regions for β1 and β3, respectively; the dynamic optimization results also indicated that the models are suitable for studies. The 3D structures and the Ramachandran plots of β1 and β3 are shown in Fig. 2A and B, respectively. Fig. 2C shows the evolution of the RMSD during the dynamic process of β1 structure.
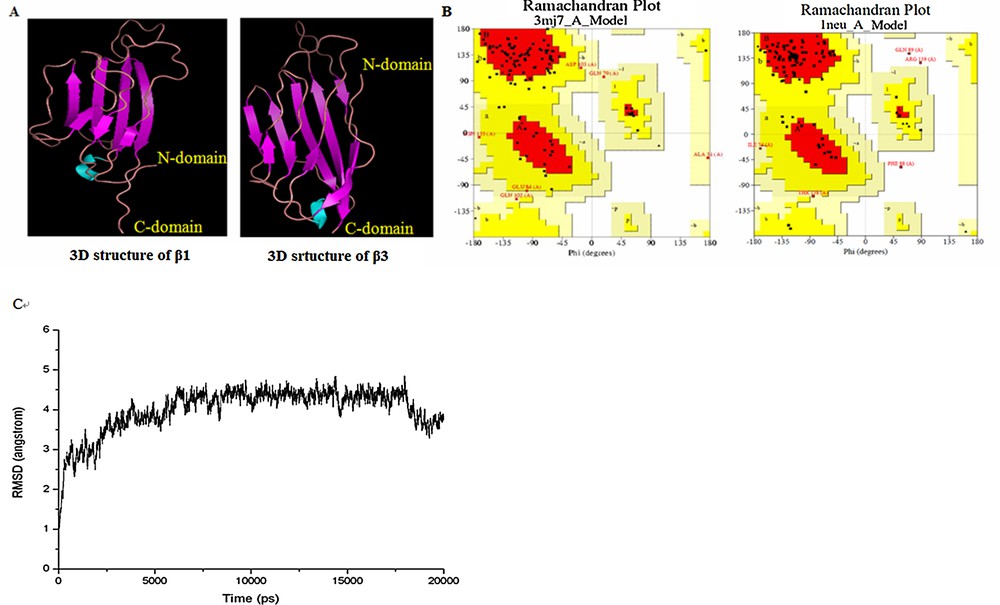
(Color online.) A. The 3D structures of β1 (left) and β3 (right) were investigated by homology modeling using 3MJ7 and 1NEU as templates. B. Ramachandran plot as the validation for homology modeling using PROCHEK. C. RMSD with respect to the simulation time for a 6-ns molecular dynamics simulation of the β1 model.
4.2 Docking study between β1 and β3
Protein–protein dockings of β1–β1 and β1–β3 were carried out (as shown in Fig. 3A and B) for studying the interaction of molecules involved in potential energy, hydrogen bond, hydrophobic contacts. The potential energies were –593.32725 (kcal/mol) and –1068.48017 (kcal/mol), respectively for β1–β1, β1–β3, and showed that the β1–β3 heterogeneous di-polymer form was more stable than the β1–β1 homologous di-polymer form (see Table 2).

A. (Color online.) The docking model of β1–β1 was shown with one pair of hydrogen bond (His 122 and Lys 140). B. The docking model of β1–β3 was shown with 10 pairs of hydrogen bonds (red for β1, blue for β3). (Glu 66----Arg 132; Glu 66----His 131; Asn 131----Pro 66; Asn 131----Arg 65; Glu 133----Arg 65; Asp 118 = ===Arg 51; Glu 120----Val 25; Ser 137----Thr 138; Asn135----Thr 138). (For interpretation of references to color, see the online version of this article.). Masquer
A. (Color online.) The docking model of β1–β1 was shown with one pair of hydrogen bond (His 122 and Lys 140). B. The docking model of β1–β3 was shown with 10 pairs of hydrogen bonds (red for β1, blue for ... Lire la suite
Molecular interaction parameters between β1 and β3 subunit.
Items | β1–β1 | β1–β3 |
Force field | CHARMm | CHARMm |
Potential energy (kcal/mol) | –593.32725 | –1068.48017 |
VDW energy (kcal/mol) | –134.90669 | –402.55395 |
Electrostatic energy (kcal/mol) | –458.42056 | –665.92622 |
Hydrogen bonding (pairs) | 1 | 10 |
Residues involved in hydrophobic contacts | a: Ser137 Thr64 Thr136 Cys43 Asn135 b: Leu39 Leu127 Phe67 Phe128 Val139 c: Glu29 Glu66 Glu133 Glu145 d: Lys44 Lys62 Lys141 Arg45 Arg46 His143 |
Thr64 Thr136 Leu117 Leu127 Leu140 Pro133 Pro142 Phe126 Phe129 Glu65 Glu67 Asp130 Arg144 |
The hydrogen bond of β1–β1 is located at 122His–140Lys, while in the case of β1–β3 ten pairs of hydrogen bonds exist: Glu 66–Arg 132; Glu 66–His 131; Asn 131–Pro 66; Asn 131–Arg 65; Glu 133–Arg 65; Asp 118–Arg 51; Glu 120–Val 25; Ser 137–Thr 138 and Asn135–Thr 138.
5 Discussion
Currently, at least four VGSC β subunits have been cloned and characterized, but little research has been done about their structure and function. Therein most of the studies focused on β1, since many mutations involving β1 have been reported in neurological diseases, such as epilepsy, EGFS+ [17].
So far, only a model of β3 structure has been elaborated by homology modelling. However, our research brought in another hypothesis using a protein–protein model for docking β1 and β3, respectively, which is more likely to form a heterodimer (β1–β3). The results based on the pairs of hydrogen bonds between β subunits and including numbers of hydrophobic groups and potential energy as an important index of the investigation were considered as well. Meaningfully, the position of the hydrogen bond (122His–140Lys) between β1 subunits was just located in disulfide linkage area in the topological structure where the GEFS + mutations sites are centralized. We can conclude that His122 and Lys140 may play an important role in the function of β1 subunit.
From the potential energy results (–593.32725 kcal/mol and –1068.48017 kcal/mol) respectively for β1–β1, β1–β3, and the number of hydrogen bonds and hydrophobic groups (between β1 and β3, there exist 9 more additional hydrogen bonds than in the β1–β1 case), we can clearly prove that the combination β1–β3 is more stable than β1–β1. Also, we can predict many additional functions for the amino acid within the binding areas for β1 or β3, for example, cell surface migration and adhesion or regulation of Na+ current.
Disclosure of interest
The authors have not supplied their declaration of conflict of interest.
Acknowledgements
This work was supported by the grants from National Science Foundation of China (NSFC, Grant No. 30902016 and No. 81072568).