1 Introduction
The Hymenophyllaceae is one of the most primitive families belonging to the class Filicopsida, Pteridophyta [1,2]. The central ecological niche of filmy ferns is a shady humid forest, at moderate altitudes in the tropics (1,500 to 300 m) or at comparable climatic conditions in lower altitudes in temperate hyperoceanic regions, such as Atlantic western Europe, southern Chile, south-east Australia, and New Zealand [3]. Filmy ferns are very common epiphytes in the temperate rain forest of southern Chile, and the most diverse genus is Hymenophyllum with 19 species [4]. One of the most remarkable characteristics of this fern group is that the frond, apart from the vascular tissue, is made of a single cell layer (hence the name filmy ferns). For this reason, they do not have a need for stomata [5]. Filmy ferns are poikilohydric organisms. They have a rudimentary ability to control water loss, and depend on high environmental humidity to be well hydrated [6]. There are some species of this filmy fern group that are able to tolerate considerable desiccation, and some of them even exhibit the ability to resurrect (the ability to lose more than 95% content of water, remain in this anhydrobiotic state for a period of time and survive after the rehydration). Very recently, Saldaña et al. [7], using whole plants reported a desiccation recovery after 60 minutes for Hymenophyllum caudiculatum and Hymenophyllum dentatum.
Desiccation tolerance is most frequently observed in mature seeds or pollen of higher plants, but very rarely observed in vegetative tissues [8]. Desiccation-tolerant plants fall into two main categories: fully desiccation-tolerant plants that can withstand the total loss of free protoplasmic water at any rate, and modified desiccation-tolerant plants that can only survive such a stress if water loss is slow. Plant complexity appears to influence which category a plant belongs to. All fully desiccation-tolerant plants studied to date are of the less complex groups of plants; algae, bryophytes, or lichens [9]. Modified desiccation-tolerant plants tend to be more complex (fern, fern allies, and angiosperms). Proteome differential expression [10] and sugar accumulation are well-known inducible desiccation tolerance mechanisms in vascularized desiccation-tolerant plants [11,12]. Using ecophysiological data, the Hymenophyllaceae have been recently described as a rare example of an evolutionary shift of adaptive strategy from that typical for vascular plants to that of poikilohydry typical for bryophytes [3]. It is hypothesized that in poikilohydrous filmy ferns where desiccation can occur very rapidly, desiccation tolerance is largely associated with constitutive mechanisms of cellular protection. Therefore, during a desiccation–rehydration cycle, it is hypothesized that the proteome and soluble sugars content should remain largely unchanged, with no significant variations.
The aim of the present study is to analyze the proteomic and physiological status of two filmy ferns, H. caudiculatum and H. dentatum, comparing hydrated, desiccated and rehydrated states, in order to understand the type of adaptive strategy the Hymenophyllaceae possesses with regard to desiccation tolerance.
2 Material and methods
2.1 Tissue sampling and desiccation treatments
H. caudiculatum (Mart.) var. productum (K. Presl.) and H. dentatum (Cav.) plants were collected from second-growth forest stands in the southern temperate rainforest in the Cordillera de Quillaipe, Katalapi Park. This site of collection has been extensively characterized previously [7]. A nursery area with automatic watering and shade cloth was previously established in order to maintain epiphytic filmy ferns taken from the field. Fronds detached from the fully hydrated plants were dehydrated at room temperature, at 70–80% relative humidity and moderate illumination for 24 h. For rehydration, the 24-h-dried fronds were placed in Petri dishes with distilled water for 24 h (Fig. 1).

(Color online). Desiccation and rehydration of detached fronds of Hymenophyllum caudiculatum (left panel) and Hymenophyllum dentatum (right panel). Fronds detached from fully hydrated plants were dried under 70–80% relative humidity and moderate illumination for 24 h (RWC 18%) and subsequently rehydrated for 24 h in Petri dishes with distilled water (RWC 82%).
2.2 Water content and Chlorophyll Fluorescence
RWC (Relative water content) was calculated as previously described [7], using twelve replicates. Chlorophyll fluorescence parameter Fv/Fm was registered with a modulated chlorophyll fluorimeter and was used as a way to monitor the activity of the photosynthetic apparatus deactivation and recovery during the desiccation–rehydration experiments.
2.3 Determination of sugar content
Concentration of sugars was measured by HPLC using a Sugar KS-802 column (Shodex, Showa Denko K.K., Tokyo, Japan) kept at 80 °C. The mobile phase was deionized water at 0.5 mL/min. Detection was made by refractive index (Waters Inc., Milford, MA, USA). Identification and quantification was made by triplicate, comparing the retention time and area with those of standard solutions.
2.4 Protein extraction and quantification
For proteomics analysis, three conditions were compared: hydrated (100% RWC), 24 h desiccated (18% RWC), and 24 h rehydrated (82% RWC). Starting with 2 g of fresh tissue, the total protein of each sample was extracted. Briefly, samples were ground in liquid nitrogen using a mortar and pestle in the presence of PVPP and precipitated with 10% TCA and 0.07% β-ME in cold acetone for one hour at −20 °C. Samples were centrifuged at 20,000 g and washed with cold acetone for one hour at −20 °C. The washing step was repeated three times. The pellets were dried at room temperature for 45 min, and then weighed and suspended in 13 μl of resuspension solution (7 M urea, 2 M thiourea, 2% CHAPS, 2% DTT, 0.2% biolyte 3/10 ampholite buffer [Biorad]) for each μg of pellet. After resuspension, the samples were centrifuged 10 min at 400 g at 20 °C, and the supernatant was transferred into a new microcentrifuge tube. Proteins were stored at −80 °C. Three extractions were completed for each sample and pooled for protein quantification. The resulting mix was quantified over four replicated assays, using the protocol described previously [13]. The mean concentration was then calculated and used to load 500 μg of proteins on each IPG strip.
2.5 Two-dimensional gel electrophoresis (2-DE)
2-DE was used to analyze the total protein content in the Hymenophyllaceae frond samples previously described. For the IEF (Isoelectrofocusing), 17-cm strips were used with a linear pH gradient ranging from 4 to 7. We have previously investigated the 3–10 pH range, without obtaining any information in the 7–10 pH range (data not show). Proteins were mixed with the resuspension solution. The IEF Protean Cell (Biorad, USA) system was programmed for active rehydration for 12 h at 50 V, then paper wicks covering the electrodes were placed and IEF resumed 1 h at 200 V, 1 h at 500 V, 1 h at 1000 V, 3 h at 10,000 V, and finally at 10,000 V per hour to achieve a total 50,000 V/h. After approximately 22 h of IEF, the strips were equilibrated 10 min with 5 ml of equilibration solution (50 mM Tris HCl pH 8.8, 6 M Urea, 30% glycerol, 2% SDS, bromophenol blue). Equilibration was performed in two steps, first with 65 mM DTT, and 135 mM iodoacetamide in the second equilibration step. SDS-PAGE was performed in batches of six gels, at 80 V in a SE900 electrophoresis unit (Hoefer, USA) for 18 h. To ensure gel reproducibility, three replicates were performed for each sample, resulting in a total of 18 gels from which the two best were selected with the help of Delta 2D 4.3 (Decodon, Germany) image analysis software.
2.6 Gel staining
Coomassie brilliant blue G-250 (Merck) was used for gel staining. Gels were fixed for 2 h in a solution containing 2% phosphoric acid and 50% ethanol. After three water washings of 30 min each, the gels were placed in an incubation solution (34% methanol, 17% ammonium sulfate, 2% phosphoric acid) for 1 h, and then immersed in a staining solution (34% methanol, 17% ammonium sulfate, 2% phosphoric acid, 0.05% Coomassie blue G-250) for five days. Finally, the gels were stored in a 5% acetic acid solution before scanning and spot picking.
2.7 Image acquisition and spot detection
Stained gels were digitalized using the Epson perfection V700 Photo Scanner and Silver Fast SE scanner software (Lasersoft Imaging AG, Germany). All the gel pictures were saved as .tif files. Image analysis was performed using Delta 2D 4.3 software (Decodon, Germany). Warping was used to eliminate local run distortions of the gels, then a fused image was created and the detected spots were transferred to all the gels. Normalized volumes were finally obtained using the normalization procedure of Delta 2D.
2.8 Statistical analyses
For H. caudiculatum or H. dentatum, one way-ANOVA was used, to test the desiccation effect (hydrated, desiccated, rehydrated) on protein accumulation in filmy fern fronds. ANOVAs were performed using Delta 2D. A protein was classified as differentially expressed between the different treatment samples if its P-value for the desiccation effect was less than 0.01. Normalized volume data for the differentially expressed proteins was analyzed using hierarchical clustering and Euclidean distance on Expander Software [14].
2.9 In-gel protein digestion
Coomassie brilliant blue-stained protein spots were manually excised from the gels and washed twice with ultra-pure water. Spots were subsequently washed in H2O/MeOH/acetic acid (47.5:47.5:5) until distaining was completed. The solvent mixture was removed and replaced by ACN. After shrinking of the gel pieces, ACN was removed and gel pieces were dried in a vacuum centrifuge. Gel pieces were rehydrated in 10 ng/μL trypsin (Sigma) in 50 mM NH4HCO3 and incubated overnight at 37 °C. The supernatant was removed and stored at −20 °C, and the gel pieces were incubated for 15 min in 50 mM NH4HCO3 at room temperature under rotary shaking. This second supernatant was pooled with the previous one, and a H2O/ACN/HCOOH (47.5:47.5:5) solution was added to the gel pieces for 15 min. This step was repeated once. Supernatants were pooled and concentrated in a vacuum centrifuge to a final volume of 30 μL. Digests were finally acidified by addition of 1.8 μL of acetic acid and stored at −20 °C. Peptide mixtures were analyzed by online capillary HPLC nanospray ion trap MS/MS (LC Packings, The Netherlands, Thermo-Finnigan, USA). Peptides were separated on a 75 μm id × 15 cm C18 PepMapTM column. The flow rate was set at 200 nL/min. Peptides were eluted using a 5–50% linear gradient of solvent B in 30 min (solvent A was 0.1% formic acid in 5% ACN, and solvent B was 0.1% formic acid in 80% ACN). The mass spectrometer was operated in positive ion mode at a 2.5 kV needle voltage and a 44 V capillary voltage. Data acquisition was performed in a data-dependent mode consisting of alternatively in a single run, a full scan MS over the range m/z 50–2000 and a full scan MS/MS in an exclusion dynamic mode. MS/MS data were acquired using a 2 m/z units ion isolation window, a 35% relative collision energy, and a 5-min dynamic exclusion duration. Peptides were identified with Proteome Discoverer 1.3. Since the Hymenophyllaceae genome has not been sequenced, peptide sequences from MS/MS data were matched to the non-redundant protein sequence database (34,337,076 entries), using peptide BLAST program blastp with the following parameters, recommended for matching short amino acid sequences: exception value 20,000, do not filter low complexity regions, gap opening const = 9, gap extension cost = 1, protein scoring matrix PAM30, word size 2.
3 Results and discussion
3.1 Relative water content and Fv/Fm during desiccation–rehydration cycle
H. dentatum tended to desiccate faster than H. caudiculatum (Fig. 2), although the final level of hydration reached was the same in both species. Similarly, Fv/Fm decreased faster in H. dentatum than H. caudiculatum. Both filmy fern species recovered their hydration state reaching RWC of 80% in about 2.5 hours, H. dentatum recovered to a hydration state of about 80% RWC rather quickly in 20 min upon rehydration, while H. caudiculatum needs almost 2 hours to reach the same level of hydration. Fv/Fm remained low about 0.5–0.6 for both filmy ferns species, and therefore there was no total recovery of photosynthetic function after 2.5 hours of rehydration. There is no clear indication that these species have different desiccation tolerances at least in the present experimental set-up. This is consistent with previous results [7] where the RWC at which photo-inactivation initiates and the level of recovery of these two species did not significantly differ. The rapid desiccation and recovery in H. caudiculatum, and H. dentatum is consistent with earlier findings, suggesting that in general for Hymenophyllaceae, the pattern of desiccation tolerance is similar to that seen in bryophytes [15,16]. Recovery of photosynthesis in bryophytes after desiccation can be remarkably rapid, requires only limited chloroplast protein synthesis and is substantially independent of protein synthesis in the cytoplasm [17]. The Hymenophyllaceae appears to recover quickly and fully after desiccation for a week or two, and within limits these species seem well adapted to cope with alternating wet and dry periods [18]. Our results are valid for detached fronds, whole plants may produce different results with longer desiccation times.

Relative water content (RWC) and Fv/Fm for Hymenophyllum caudiculatum and Hymenophyllum dentatum, during desiccation and rehydration during 160 min. Means ± s.d., n = 12.
3.2 Sugar accumulation
In order to establish if sugar accumulation was induced by a desiccation–rehydration cycle, sugar content was measured in H. caudiculatum and H. dentatum, in hydrated, desiccated (24 h) and rehydrated (24 h) fronds. For both species only sucrose was detected by HPLC–DAD. The accumulation of soluble sugars has long been correlated with the acquisition of desiccation tolerance in plants and other organisms [19]. One function may protect the cell via glass formation [20], a liquid with the mechanical properties of a solid. Secondly, sucrose may maintain hydrogen bonds within and between macromolecules and maintain the structure [21]. H. caudiculatum fronds showed no significant differences between hydrated (137 ± 9 μmol g−1 DW) and desiccated (129 ± 39 μmol g−1 DW) states, and a decrease in rehydrated (66 ± 9 μmol g−1 DW) fronds. In H. dentatum sucrose increased from hydrated (205 ± 24 μmol g−1 DW) to desiccated (279 ± 13 μmol g−1 DW) and decreased in rehydrated state (175 ± 14 μmol g−1 DW). These results are in contrast with the increase of sugars observed in higher plants. In the leaves of Xerophyta viscose, a monocotyledonous poikilochlorophyllic resurrection plant, the concentration of sucrose was increased 5-fold from 4.8 mg g−1 DW to 23.5 mg g−1 DW at 5% RWC. At 7 days after rewatering, the leaf sucrose concentration had decreased to levels comparable with that of the fully turgid state [22]. Furthermore, in leaves of Sporobolus stapfianus, a poaceae the mean rate of sucrose accumulation was calculated for RWC interval periods of 84–75%, 75–68%, and 68–42%, in which the accumulation was reported to be significant with values of 25, 80 and 175 μmol g−1 DM [23]. On the other hand, sucrose makes up approximately 10% of the dry mass of Tortula ruralis gametophytes and does not change in amount during desiccation or rehydration in the dark or light [24]. The lack of an increase in soluble sugars in response to desiccation appears to be a common feature of tolerant mosses [25]. Thus, it appears important to maintain a constant, and presumably sufficient, amount of this sugar in this moss. In H. dentatum and H. caudiculatum, sucrose content was maintained between (5.6–9.2%) and (1.9–7.0%) of the dry mass, respectively.
3.3 Proteomics distance between desiccation states
A two-dimensional gel electrophoresis was used to analyze the proteome variation of Hymenophyllaceae fronds comparing hydrated, desiccated, and rehydrated states. A reference 2-DE map was established using the proteins extracted from hydrated, desiccated, and rehydrated fronds (Fig. 3). For H. caudiculatum and H. dentatum, a total of 731 and 802 spots respectively were placed on the reference map that was further used to compare the abundance of each spot between the three conditions. Comparative analysis of the 2-DE images showed that the proteome patterns were similar in the three 2-DE images, indicating that most of the proteins showed similar amounts between hydrated, desiccated and rehydrated states (Supplementary Fig. S1). Statistical analysis using ANOVA allowed the detection of 112 spots in H. caudiculatum and 91 spots in H. dentatum, each showing significant differences between the three conditions. The clustering of the significant 112 and 91 spots for both species analyzed in this study clearly showed that rehydration was the main factor controlling protein accumulation in H. caudiculatum and H. dentatum fronds (Fig. 4). Indeed, for both species, technical replicates clustered together, then hydrated and desiccated samples and finally rehydrated samples. The same conclusions could be drawn from the analysis of the overall dataset (data not shown). A simple pairwise comparison (t-test) confirmed that the transition from desiccated to rehydrated fronds presented the most important effect on protein abundance. Indeed, for H. caudiculatum and H. dentatum, only a 3% or 4.1% of detected proteins exhibited distinctive accumulation patterns between hydrated and desiccated states, in contrast to 10.4% and 8.7% of proteome variation between desiccated and rehydrated fronds. Previous proteomic studies in Selaginella bryopteris identified 250 reproducibly detected proteins, and 19.2% showed significant and reproducible changes in abundance [26]. In Boea hygrometrica, 223 proteins were reproducibly detected, and the abundance of 54% changed with desiccation [10].
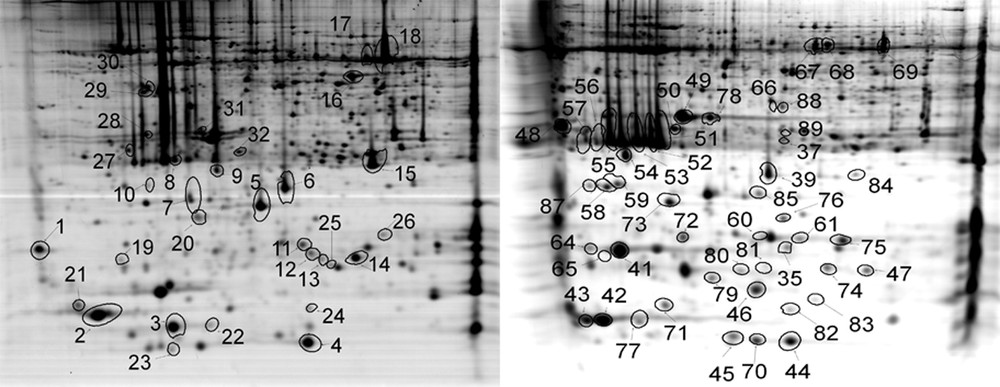
Two-dimensional gel electrophoresis (2D-E) maps of Hymenophyllum caudiculatum (left) and Hymenophyllum dentatum (right). Proteins that were identified are marked with circles and numbered as in Supplementary Table S1.
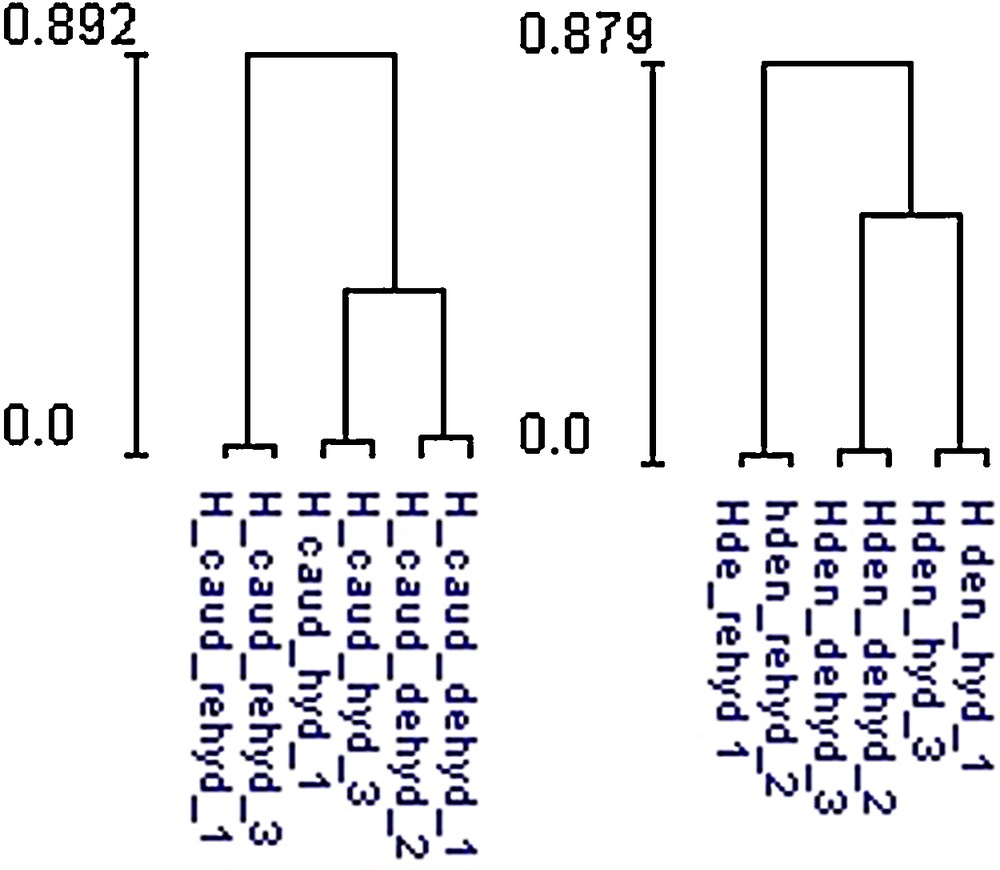
Clustering result between hydrated, desiccated and rehydrated samples for Hymenophyllum dentatum and Hymenophyllum caudiculatum, based on significantly expressed spots between the three conditions.
3.4 Protein identification success rate
A total of 1417 spots were placed in both reference maps from which 82 (5.8%) were excised from the polyacrilamide gel and analyzed by mass spectrometry. As show in Fig. 3, spots were picked randomly to ensure a good representation in terms of pH, molecular weight and protein abundance. LC ESI MS/MS of 82 spots was used for protein identification using protein and nucleotide databases (Supplementary data). The overall identification success rate was 79%, corresponding to 65 proteins identified.
3.5 Protein identification and functional classification
A summary of protein function is given in Supplementary Table S1. Proteins were classified into seven groups based on functional categories using the MIPS system (http://mips.helmholtz-muenchen.de/proj/funcatDB/search_main_frame.html) [27]. Major groups were energy-photosynthesis (33 spots, 51%), Hypothetical proteins (18 spots, 28%), and cell rescue defense and virulence (5 spots, 8%). Overall, 73% of the identified proteins appeared as multiple spots, and photosynthetic enzymes dominated the identified spots: light-harvesting complex included (proteins # 9, 34, 54, 48, 58, 64), chlorophyll a/b binding protein (proteins # 51, 52, 53, 55, 56, 57, 8, 19, 10, 39), photosystem I subunit VII (proteins # 4, 5, 12, 23, 44, 45), oxygen-evolving enhancer protein 1 (proteins # 50, 78, 49, 31), ribulose-1,5-bisphosphate carboxylase/oxygenase large subunit (proteins # 17, 18, 69, 70), energy regeneration proteins; ATP synthase CF1 alpha subunit (proteins # 67, 68), detoxification related; 2-cys peroxiredoxin (proteins #7, 87), and unclassified proteins: 14-3-3 protein (proteins # 27, 28), Hypothetical protein SELMODRAFT_4375 (proteins # 61, 35). The multiple spots identification may reflect posttranslational modification, allelic variation of the same protein, isozyme variation, alternative splicing events or protein degradation.
The relatively high concentrations of the abundant photosynthetic enzymes demonstrate the importance of these enzymes; however, the prominence of these proteins, specifically chlorophyll a–b binding proteins, in specific regions of the gel, contributes to lower quality 2-DE gels and prevents the observation of moderate or lower abundance proteins due to their relatively lower concentrations and the limited dynamic range of common 2-DE staining techniques including Coomassie colloidal blue [28].
3.6 Energy metabolism response to desiccation and rehydration
Photosynthesis is a major characteristic metabolic process of green plants. In H. dentatum fronds, a group of six protein species of apparent molecular weight about 29.5 kDa and isoelectric point between 4.75 and 5.18 (Fig. 5A–C) were identified as Chlorophyll a/b binding proteins in proteins #57, 56, 55, 53, 52 and 51. Light-harvesting chlorophyll a or b binding proteins function in the collection and transfer of light energy to the reaction centers of photosystem II (PSII) (Lhcb proteins) and photosystem I (PSI) (Lhca proteins). Additionally these proteins are involved in light dissipation and energy quenching. Besides the large number of lhc gene products, posttranslational modifications, such as phosphorylation, contribute to greater complexity at the protein level [29]. Protein numbers #57 and #56 were accumulated in hydrated state (Fig. 5A), and declined in the desiccated (Fig. 5B), and rehydrated (Fig. 5C) states. This may be related to posttranslational modification of the protein species, related proteins #52 and #51 increased their accumulation, saturating the spot region in desiccated and rehydrated states. Oxygen-evolving enhancer protein 1 is required for photosynthetic oxygen evolution [30]; this protein was identified in three spots (Fig. 5A–C) where #49 and #50 remained constant in all conditions, spot #78 was increased during desiccation and then decreased during dehydration. Proteins #49 and #78 have the same apparent molecular weight and showed altered mobility, which may have been due to posttranslational modifications. The large subunit of Rubisco (Protein#18 for H. caudiculatum, #69 for H. dentatum) remained highly abundant in hydrated, desiccated and rehydrated fronds (Fig. 5D–F). ATP synthase CF1 alpha subunit, (Protein#67 and #68 in H. dentatum) participates in the ATP generation in the thylakoid membrane, the accumulation of this protein species was constant between hydrated, desiccated or rehydrated fronds (Supplementary Fig. S1). Proteomic analysis of the photosynthetic proteins in hydrated, desiccated and rehydrated states showed that in H. dentatum and H. caudiculatum, photosynthetic structures are well preserved during desiccation (Homoiochlorophyllous), which may enable this plant to effectively rapidly recover its photosynthesis capacity.
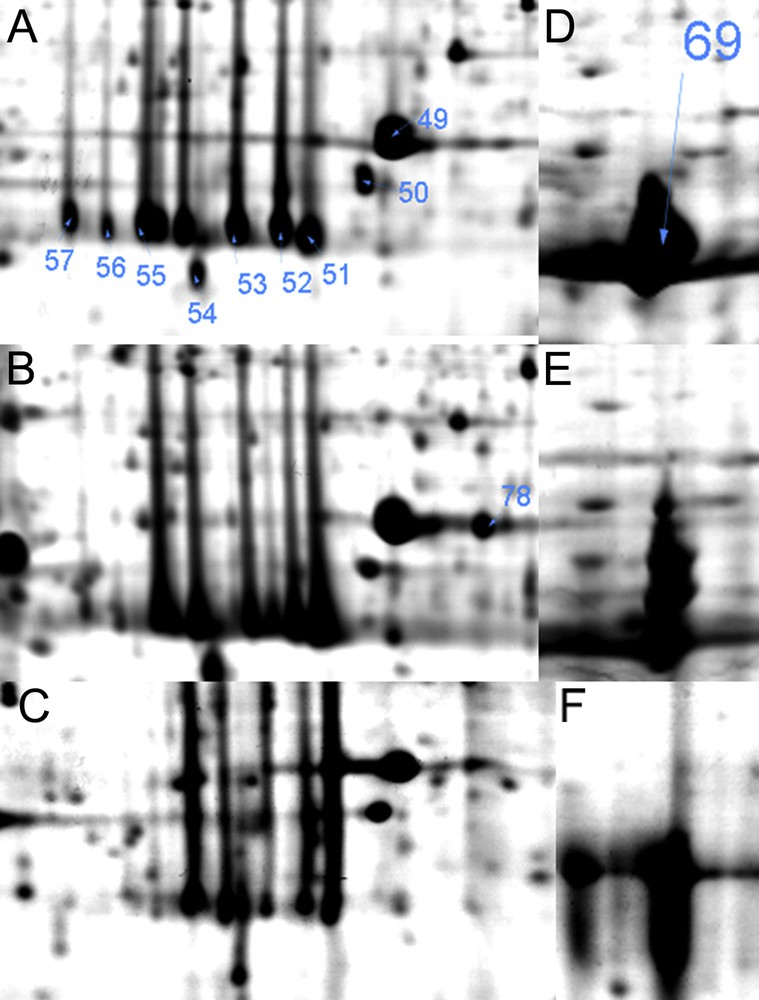
Magnified views of photosynthesis related proteins in Hymenophyllum dentatum in response to different desiccation states A and D (hydrated); B and E (desiccated); C and F (rehydrated). Arrows indicate proteins listed in Supplementary Table S1.
3.7 Antioxidant system response to desiccation and rehydration
During the course of photosynthesis and respiration, cells continuously produce O2 intermediates such as superoxide radical, hydrogen peroxide, and hydroxyl radicals, commonly known as ROS. Two 2-cys peroxyrredoxin proteins were identified, #7, in H. caudiculatum, was highly accumulated (volume expression 0.38–0.86) in hydrated and rehydrated fronds, while protein # 87 in H. dentatum tended to be more most accumulated in desiccated fronds. Peroxiredoxins, also termed thioredoxin peroxidases, are a family of thiol-specific antioxidant enzymes that are critically involved in cell defense and protection from oxidative damage [31]. One Cytosolic copper/zinc superoxide dismutase variant 1 of H. caudiculatum was more abundant in desiccated fronds (protein #25), while one pyridoxal biosynthesis protein (superoxide-inducible protein 7) (#88) was accumulated in desiccated fronds of H. dentatum. In modified desiccation-tolerant plants (including many vascularized resurrection plants), tolerance depends on protective mechanisms set in place during slow drying, with more or less extensive repair of cell damage following rehydration. In fully desiccation-tolerant plants (e.g., T. ruralis), recovery depends on a combination of constitutive tolerance, and repair takes place during and following rehydration [32].
4 Conclusion
This research examined the adaptative strategy of two species from the Hymenophyllaceae family for desiccation tolerance, analyzing the physiological and proteomic responses in two species of the filmy ferns, H. caudiculatum and H. dentatum. Physiological data showed a good correlation with the proteomic analysis. Both species of the Hymenophyllaceae show a pattern of poikilohydric homoiochlorophyllous adaptation, which they share with the bryophytes that grow in profusion in the same habitats. The speed at which desiccation takes place in these two species precludes the induction of protective systems that require time for transcription of specific gene sets and the translation of the resulting transcripts into active proteins. This strategy is consistent with the short-term nature of alternating wet and dry periods (Proctor, 2003). While the present study is a good starting point for understanding the desiccation tolerance in these filmy fern species, further investigation is needed to determine the role of unknown proteins in the mechanisms of constitutive cellular protection and of the rehydration-induced recovery process that is presumably designed for the repair of cellular damage following a desiccation stress.
Disclosure of interest
The authors declare that they have no conflicts of interest concerning this article.
Acknowledgments
Prof. Carolina Shene De-Vits is gratefully acknowledged for the analysis of sucrose content. We thank Charles Guy for his review and for improving the English. This research was supported by a post-doctoral grant FONDECYT 3120195 and PIA-UFRO DI10-7006.