1 Introduction
Heteroptera are one of the most important groups of insects because of their adaptation capacity, and ecological success as well as vectoring several disease agents to plant [1]. These insects commonly known as true bugs or stinkbugs represent over 38,000 described species, including Eurygaster integriceps Puton (Scutelleridae) with its piercing–sucking mode of feeding [2]. This kind of feeding is typical of heteropterans, piercing and cutting tissues with their stylets while injecting digestive enzymes through the salivary canal to pre-digest food, a process that is also known as pre-oral digestion [3]. Then pre-digested food is ingested through the food canal and passes into the alimentary canal, where it is further digested by digestive enzymes and absorbed [4,5].
Pre-oral digestion is important for those bugs feeding on seeds and prays, but not for sap suckers. Some of the advantages of this feeding strategy for predaceous bugs are: (1) the ability to circumvent the feeding barrier, cuticle; (2) the ability to bypass defensive chemicals; and (3) the ability to use of larger prey [6]. Similar benefits can also be hypothesized for pre-oral digestion in phytophagous bugs, especially seed-sucking ones. These heteropterans feed from hard plant seeds that are covered by a cuticle. Besides, plant seeds are generally containing different defensive chemicals, like enzyme inhibitors and antifeedants [7]. Thus, it is not possible for the bugs to feed from hard soiled seed material without liquefying it. Finally, these insects test the host plant's suitability by a probe that is based on pre-oral digestion. Taken together, the evidences highlight the importance and benefits of pre-oral digestion in phytophagous heteropterans like E. integriceps.
The salivary glands of most insects are labial glands that produce saliva, which is responsible for mouthpart lubrication and initial digestion of food sources [1]. Although considerable numbers of Heteroptera are sap-suckers, a majority of them, however, feed on pray or seeds. Therefore, salivary glands digestive enzymes play important roles in the pre-digestion process of the insects. It has been suggested that these insects have a relatively complete profile of enzymes in their salivary glands to be used in pre-oral digestion [8]. Salivary glands of Pentatomorpha produce two types of saliva. The sheath-forming saliva (gelling saliva), which is secreted by the anterior lobe of salivary glands, and the watery saliva, secreted by the posterior lobe [8]. Watery saliva is the major salivary gland secretions containing digestive enzymes. Silva and Terra [9] detected enzymatic activities in saliva of Dysdercus peruvianus (Pyrrhocoriade); however, the levels of enzymatic activities in the salivary glands were less than those in the midgut. Boyd [10] showed that the salivary glands of Deraeocoris nigritulus (Miridae) contained trypsin, chymotrypsin, and pectinase. The presence of different salivary digestive enzymes has been reported for other bugs, e.g., protease and amylase in giant water bugs (Belsomatidae) [11], proteinase (collagenase) Podisus nigrispinus (Pentatomidae) [12] and pod-sucking bugs (Coreoidae) [13]. These reports suggest that almost all of heteropterans produce digestive enzymes in their salivary glands. Conflicting suggestions have been reported, however, about the role of salivary enzymes in the digestive process of heteropterans. Some of them reported the important role of digestive enzymes in pre-oral digestion of heteropterans [3–5,10,14]. Others, in contrast, proposed no digestive role for salivary enzymes in heteropterans bugs [9].
Considering the importance of pre-oral digestion in the feeding process of heteropterans, especially seed feeders, study of digestive enzymes as an important component of this process deserves more attention. The results arising from such studies would provide useful information on digestion in insects and insect–host plant interactions. Therefore, the present work aimed to characterize digestive enzymes in SGC of E. integriceps and their response to starvation and the feeding period. It is showed that salivary glands of this insect contain a relatively complete set of digestive enzymes which were induced in response to feeding. It was also revealed that salivary glands produced several isoform of amylases with different biochemical properties.
2 Material and methods
2.1 Insect rearing
A number of 100 individuals of E. integriceps were used in this study. Female adults of E. integriceps were obtained from laboratory cultures. Insects were placed in containers (plastic dishes) with access to water and without feeding, in laboratory conditions at 25 ± 2 °C and a photoperiod of 14:10 (L: D) for 10 days. Then, the starved insects were allowed to feed on wheat kernels for 3 hours Then, a number of 20 insects were selected and dissected as described in the following section. Starved insects (20 insects) were also dissected and considered as controls.
2.2 Insect dissection and enzyme preparation from salivary gland complexes (SGC)
Adult females were individually immobilized by placing them on crushed ice (8–10 min), then they were dissected under the stereomicroscope in pre-cold 215 mM NaCl, and their SGC were removed. Drawings were made with the aid of a stereomicroscope at 10× magnification.
Enzyme preparation was based on the previously described methods, with slight modifications [15,16]. Groups of pooled SGC were homogenized in distilled water by a motorized Potter–Elvehjem homogenizer (Teflon pestle, 0.1 mm clearance). Then, the SGC homogenate from starved and fed insects were transferred separately into 1.5-mL centrifuge tubes and centrifuged at 15,000 g for 30 min at 4 °C. The final volumes of both supernatant and pellet were adjusted to 1 mL by double-distilled water.
2.3 Protein determination
Protein concentration was measured according to the method of Bradford [17], using bovine serum albumin (Bio-Rad, Munich, Germany) as a standard.
2.4 Hydrolase assays
The α-amylase activity was assayed by the dinitrosalicylic acid (DNS) procedure [18], using 1% soluble starch or 1% soluble glycogen. α- and β-glucosidase activities were measured using 5 mM of 4-nitrophenyl α-d-glucopyranoside and 4-nitrophenyl β-d-glucopyranoside (NPα/βGlu), respectively, in a 50 mM citrate–phosphate buffer at pH 5.0, based on the appearance of p-nitrophenol in the solution according to Terra et al. α-/β-Galactosidase activities were assessed using 5 mM of 4-nitrophenyl α-d-glucopyranoside and 4-nitrophenyl β-d-glucopyranoside (NPα/βGal), respectively, in a 50 mM citrate–phosphate buffer at pH 5.0. α- and β-manosidase activities were measured using 2.5 mM of 4-nitrophenyl α-d-manopyranoside and 4-nitrophenyl β-d-manopyranoside (NPα/βMan), respectively in a 50 mM citrate–phosphate buffer at pH 5.0. Lipase activity was measured using 1 mM of p-nitrophenyl butyrate (pNPB) in a 50 mM citrate–phosphate buffer at pH 5.0. Aminopeptidase activity was measured using 2 mM l-leucine p-nitroanilide (LpNA) in 50 mM of a Tris–HCl buffer at pH 7.0 as a substrate [19]. The activity of acid phosphatase was determined using 5 mM of 4-nitrophenyl phosphate (pNPP) in 50 mM of a citrate–phosphate buffer at pH 4.5 as a substrate. Alkaline phosphatase was determined using 50 mM of a glycine–NaOH mixture at pH 10.4 containing 1 mM of MgSO4 [20]. General proteolysis was done according to the methods of Elpidina et al. [21] and Gatehouse et al. [22] using 2% azocasein in a Tris–HCl buffer at pH 8.0. Trypsin- and chymotrypsin-like activities were assayed using 1 mM of N-benzoyl-l-arginine-p-nitroanilide (BApNA) and 1 mM of N-succinyl-l-Ala-l-Ala-l-Pro-Phe-p-nitroanilide (SAAPFpNA) [21,22]. The substrate was dissolved in dimethyl sulfoxide (DMSO) and then diluted in a buffer so that the final concentration of DMSO in solution was less than 10%.
The hydrolysis of the substrates was monitored continuously at 410 nm at 30 °C and initial rates were measured from the slopes of absorbance against time. For each determination, the mixture of the reagents was kept at 30 °C for at least four different periods of time, and initial velocities were calculated. Controls without enzyme or substrate were carried out and treated in a similar way to the experimental groups. The enzyme activity was expressed in milli units (mU). One unit of enzymatic activity was defined as the amount of enzyme that hydrolyzes 1 mmol of substrate per minute.
The pH optima of enzymes were determined using a universal buffer [23]. The pH values tested were 3, 4, 5, 6, 7 and 8.
2.5 Kinetic studies
The dependence of the hydrolytic activity of the amylase on substrate concentration was analyzed. The assay was performed in the presence of substrate concentrations of 0.05, 0.1, 0.2, 0.4, 0.6 and 0.8% of starch or glycogen, but the enzyme concentration was kept constant. Controls were run in parallel, in which distilled water replaced the enzyme for each substrate concentration. Double reciprocal plots (Lineweaver–Burk plots) of enzyme activity versus substrate concentration were used to establish that Michaelis–Menten kinetics were obeyed over this substrate concentration range and to obtain values of the maximum velocity (Vmax) and Michaelis constant (KM).
2.6 Ammonium sulfate precipitation and gel filtration chromatography
The crude extract (40 mL) from 100 salivary glands homogenates of E. integriceps adults was treated with ammonium sulfate at 4 °C to give fractions precipitating at 20%, 30%, 40%, 50%, 60%, and 80% saturation. The precipitates were collected by centrifugation at 10,000 g for 15 min, diluted in 2 mL of a 0.02 mol/L phosphate buffer (pH 6.5) and dialyzed overnight at 4 °C against the same buffer. The enzyme solution was applied to a Sephadex G-75 column (1.5 cm × 80 cm), equilibrated with a 0.02 mol/L phosphate buffer (pH 6.5). The column was run at a flow rate of 0.5 mL/min and 1.5 mL fractions were collected. The protein level of each fraction was monitored at 280 nm and amylase activity was measured as described in the previous section. Fractions with enzymatic activity were pooled and concentrated by lyophilization. The lyophilized powder was dissolved in the least amount of phosphate buffer and applied to a diethylaminoethyl (DEAE)-cellulose column (1.3 × 28 cm), equilibrated with a 0.02 mol/L phosphate buffer (pH 6.5). The enzyme elution was done at a flow rate of 0.5 mL/min with a linear NaCl gradient (0–0.6 mol/L). Fractions (1.0 mL/tube) were collected and their protein concentration and α-amylase activity were determined as described.
2.7 Native PAGE
To detect the amylolytic activity of SGC, native SDS-PAGE was carried out. Native PAGE was performed in 10% (w/v) gel with 0.05% SDS for separating the gel from the stacking gel (5%) with 0.05% SDS. After electrophoresis, the gel was rinsed with distilled water and washed by gentle shaking in 1% (v/v) Triton X-100 dissolved in a phosphate buffer containing 2 mM of CaCl2 and 10 mM of NaCl for 30 min Then the gel was rinsed with distilled water and treated with a 1% starch solution for 1.5 h. Finally, the gel was treated with a solution of 1.3% I2, 3% KI to stop the reaction and to stain the unreacted starch background. Zones of α-amylase activities appeared as a light band against the dark background of the gel.
2.8 SDS-PAGE
To have an insight into the protein contents of SGC, SDS-PAGE was applied. The acrylamide concentration was 10% for the separating gel and 4% for the stacking gel, and proteins on the polyacrylamide gel were stained with 0.2% of Coomassie brilliant blue R-250. β-Galactosidase (116 kDa), bovine serum albumin (66.2 kDa), ovalbumin (45 kDa), lactate dehydrogenase (35.5 kDa), restriction endonuclease Bsp 981 (25 kDa), β-lactoglobulin (18.4 kDa) and lysozyme (14.4 kDa) were used as molecular mass standards.
2.9 Data analysis
Data were compared by one-way analysis of variance (ANOVA) followed by LSD test. When P < 0.01, the differences were considered to be significant. To compare means of enzymatic activities before and after feeding, a paired-Student's t-test was also used.
3 Results
3.1 Anatomy of SGCs from E. integriceps
The SCGs of E. integriceps occur in pairs, just around the digestive canal, and the glands extend back into the thorax. The SGCs consist of branching short tubules fusing together at the center of the salivary gland, producing concentric semicircles. Each tubule usually divides and forms a branched structure (Fig. 1). The glands are bathed in the hemocoel. The SGCs are composed of two sections, the principal gland (PG) and the accessory gland (AG) (Fig. 1). The principal glands are further divided into two lobes, which are referred to as anterior lobe (AL) and posterior lobe (PL). The shape of the posterior and anterior lobes is the same; the posterior lobe, however, is about 2 times bigger than the anterior one and is the main SGC. The accessory gland is an elongate tubular structure, fusing to the principal glands. The principal and accessory glands have their own ducts and these ducts lead to the lateral salivary duct. The lateral ducts from each side fuse to form a median salivary duct. The tracheoles are quite extended on the salivary glands surface. The salivary glands were reported to be acidic using chemical indicators (pH: 5.5–6.0) [7]. There was no difference in pH between salivary glands from starved and fed insects using chemical indicators (data not shown).

Schematic drawing of the gross morphology of the salivary gland complexes (SGC) of E. integriceps. The SGC are composed of two sections, the principal gland (PG) and the accessory gland (AG). The principal glands are further divided into two lobes, which are referred to as anterior lobe (AL) and posterior lobe (PL). LD: lateral duct; PD: principal duct.
3.2 Digestive enzymes of SGC
The extents of the enzymatic activities of SGC were dependent on the pH value of the assay medium (Fig. 2). Despite α-glucosidase with an optimum pH at 8.0, the highest carbohydrase activities were seen at pH 5.0–6.0 (Fig. 2). The activities dropped markedly outside the narrow optimum pH at 30 °C. Lipase had an optimum activity at pH values between 7.0 and 8.0, while phosphatase showed two pH optimum activities at 5.0 and 9.0 (Fig. 2).
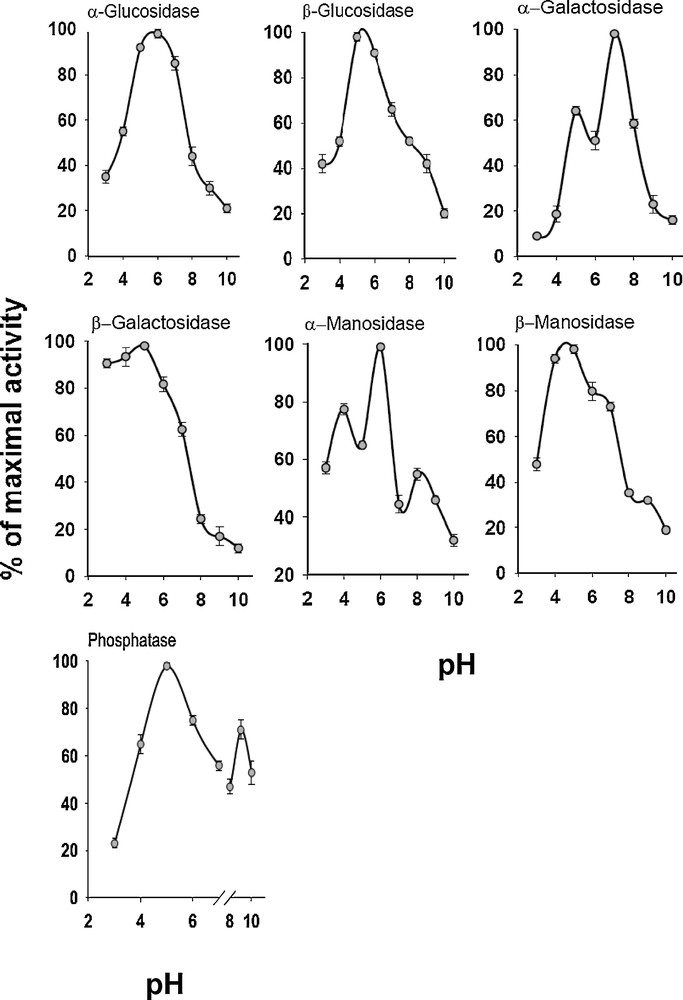
Effect of pH on the relative activities of the digestive enzyme extracted from SGC of an adult individual of E. integriceps. Optimal pH for each enzyme activity was determined using an universal buffer (glycine 0.02 M, succinate 0.02 M, 2-(N-morpholino)ethanesulfonic acid, MES, 0.02 M) whose pH was adjusted by NaOH 1.0 N. The bars represent the SD (n = 5 replicates).
Investigation of the profile of digestive enzymes of the salivary glands using general and specific substrates evidenced the major enzymatic activities in PG (especially PL) (data not shown). Activities of some enzymes in PL, however, were not high enough to be detected readily; then, whole SGC extract was used. Enzymatic activities against almost all the substrates used were found (Fig. 3). Results showed that salivary α-amylase had higher activity against starch than glycogen (Fig. 3A). Carbohydrases were detected in SGC with different activities; the largest carbohydrase activities were recorded for α/β-glucosidases. Enzymatic activities toward mannose (α/β-mannosidases) were low in comparison to activities against glucose (α/β-glucosidases) and galactose (α/β-galactosidases) (Fig. 3B–D). Both alkaline and acid phosphatases were detected in SGC using buffers with different pHs. However, acid phosphatase showed a seven-fold higher activity than alkaline phosphatase (Fig. 3E). We detected no measurable activities for lipase and aminopeptidase. Proteolytic activity against hemoglobin was higher than that against azocasein (Fig. 3F). Moreover, trypsin activity was higher than chymotrypsin activity in the salivary glands, as detected by specific substrates (Fig. 3G).
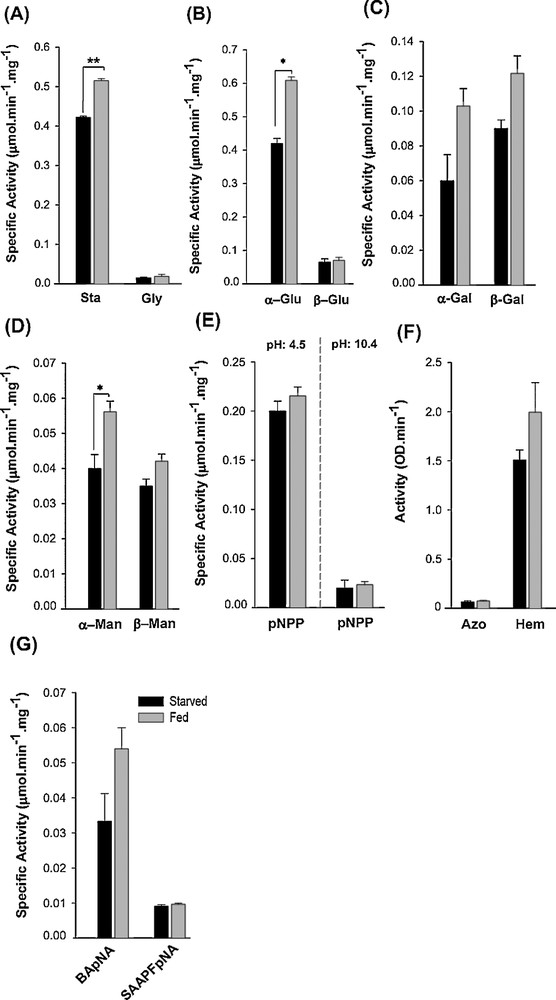
Comparison of the activities of the digestive enzyme in the SGC of an adult individual of E. integriceps and their response to starvation and feeding. The bars represent the standard errors across triplicate samples. The enzymatic activities in starved and fed insects, marked by (*) and (**), respectively, were significantly different when determined by Student's t-test (P < 0.05 and P < 0.01, respectively). Sta: starch; Gly: glycogen; α-Glu: α-glucosidase; β-Glu: β-glucosidase; α-Gal: α-galactosidase; β-Gal: β-galactosidase; α-Man: α-mannosidase; β-Man: β-mannosidase; pNPP: 4-nitrophenyl phosphate; Azo: azocasein; Hem: hemoglobin; BApNA: N-benzoyl-l-arginine-p-nitroanilide; SAAPFpNA: N-succinyl-l-Ala-l-Ala-l-Pro-Phe-p-nitroanilide.
3.3 Induction of salivary digestive enzymes in response to feeding
To test the hypothesis that enzymatic activities were changed in response to starvation and feeding, investigation was done in starved and fed insects. The results showed that the activities of almost all enzymes were increased in response to feeding in comparison to starved insects (Fig. 3). Amylolytic activity against starch was increased significantly (t = 49.13, P < 0.01), while an increase in the activity against glycogen was not significant in fed insects (Fig. 3A). Despite α-glucosidase and α-mannosidase which induced significant responses to feeding (t = 37.76, P = 0.017 and t = 16.2, P < 0.05, respectively), enhanced carbohydrase activities in fed insects were not significantly higher than in starved insects (Fig. 3B–D). Acid phosphatase showed significantly higher activity in fed insects in comparison to the starved ones (t = 15.4, P < 0.05); alkaline phosphatase, however, did not change significantly (Fig. 3E). Proteolytic activities (general and specific) were increased in response to feeding, although these were not significant (Fig. 3F, G).
In complement to colorimetric assays, the SGC extract was subjected to a series of PAGE. We tested the induction of enzymatic activities in response to feeding with SDS-PAGE by using equal volumes from protein extracts of salivary glands pooled from starved and fed insects. Results showed that the intensities of the respective bands in the protein profile of the SGC of fed insects were enhanced in comparison with the starved insects’ SGC protein profile. Moreover, some new bands (less than 25 kDa) were detected only in the protein profile of the SGC of the fed insects (Fig. 4). It is noteworthy that induction of digestive amylase and protease in response to feeding was not high enough to be detected by native PAGE.

Protein profile analysis between the protein contents in SGC of starved and fed E. integriceps. SGC extract from starved and fed insects subjected to SDS-PAGE. The intensities of some bands in the protein profile of the SGC of fed insects are enhanced compared to the SGC protein profile of starved insects, as indicated by arrows.
3.4 Fractionation of SGC extracts
Considering the presence of a high α-amylase activity in SGC and also its prominent role in digestion in insects, α-amylases of SGC from E. integriceps adults were fractionated using gel filtration chromatography (Fig. 5). Each fraction was tested for amylolytic activity against starch 1% as a substrate. Fractions with high amylolytic activities were pooled as indicated in the chromatogram and subjected to PAGE analysis using the same substrate.
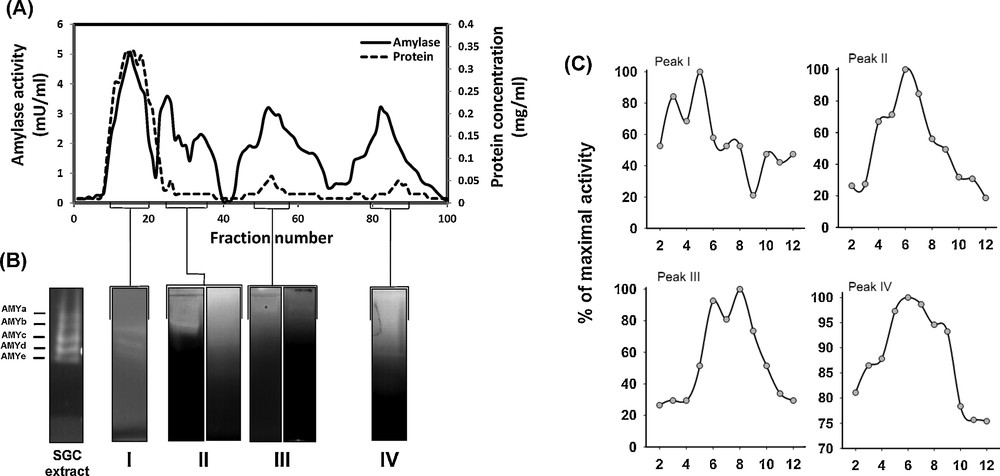
Gel filtration chromatogram of the SGC extract from an adult individual of E. integriceps. The amylase activity with 1% soluble starch was measured by spectrophotometry at 540 nm. The protein was also measured at 280 nm (A). Amylase-rich fractions (as indicated) were pooled, and then subjected to native PAGE (B), followed by the determination of optimum pH (C).
The SGC amylase activity eluted in four major peaks (noted as I to IV). The highest amylolytic activity in SGC fractions was observed in the first peak of the chromatogram with pH optima at 5.0 (Fig. 5A, C). The second peak of amylase activity showed an optimal pH value of 6.0 (Fig. 5A, C). Amylases in two other peaks (III and IV) from SGC had their maximal activities at pH values of 8.0 and 6.0, respectively (Fig. 5A, C).
In complement to colorimetric inhibition assays, the fractions were subjected to a series of non-denaturing PAGE. The post-electrophoretic detection of the starch digestion by amylase demonstrated existence of at least five isoamylases in the SGC (e.g., AMYa, AMYb, AMYc, AMYd, and AMYe) (Fig. 5B). Zymography of the four amylase-rich fractions of SGC showed that they contained different isoamylases (Fig. 5B). Since pooled fractions showed various optimum pH values as well as different isoamylases, some kinetic parameters were determined for the fractions. The results revealed that the fractions had different kinetic parameters against starch (1%) and glycogen (1%). Interestingly, it was shown that the amylases of the insect had more efficiency (e.g., Vmax/KM ratios) on starch than on glycogen (Table 1). There were significant differences among the catalytic parameters of the different fractions. Among the fractions, fraction IV and III showed the highest Vmax/KM ratios against starch and glycogen, respectively (Table 1).
Comparison of the kinetic parameters of different amylase-rich fractions obtained from gel filtration chromatography. The assay was performed in the presence of different concentrations of substrates (e.g., starch and glycogen). The maximum velocity (Vmax) and Michaelis constant (KM) were obtained graphically using a double reciprocal plot (Lineweaver–Burk plot) of enzyme activity versus substrate concentration.
Amylase-rich fractions | Starch | Glycogen | ||||
KM (%) | Vmax (μmol/min/mg protein) |
V max/ K M | KM (%) | Vmax (μmol/min/mg protein) |
V max/ K M | |
I | 0.084d | 0.003a | 0.036a | 0.081d | 0.001a | 0.012a |
II | 0.018a | 0.080c | 4.440c | 0.050b | 0.016b | 0.320c |
III | 0.055c | 0.016b | 0.290b | 0.047b | 0.018b | 0.383d |
IV | 0.018a | 0.401d | 22.28d | 0.068c | 0.019b | 0.279b |
4 Discussion
In the present study, the morphology of the SGC of E. integriceps was described, and also the activities of the digestive enzymes of the SGC as well as the induction of the digestive enzymes were studied. Morphological studies showed that SGC in E. integriceps are, as in other heteropterans salivary glands consisting of AG and PG, the latter being further divided into AL and PL. These morphological features have been reported for other phytophagous heteropterans, i.e. Clavigralla tomentosicollis, Clavigralla shadabi, Riptortus dentipes, and Mirperus jaculus [13]. Heteroptera (here E. integriceps) exhibit a very wide range in form and structure of labial glands. This variety is not surprising due to the numerous feeding habits represented in heteropteran bugs, including zoophagous, phytophagous, zoophytophagous, granivorous, and haematophagous species [8,24]. Despite this diversity, however, the overall morphology of the salivary glands is the same, i.e. a principal gland with an accessory gland on each side. The principal glands are fundamentally bi-lobed, comprising anterior and posterior lobes. The principal duct extended forward into the head, where it joins its counterpart to form a common duct that passes into the characteristic salivary pump and opens into its distal end.
We found that PL is the main source of digestive enzymes in SGC of E. integriceps. These results are in accordance with those reported for other bugs, e.g. Lygus disponsi and L. rugulipennis, i.e. that PL is the main source of digestive enzymes in salivary glands of bugs as described by Miles [8].
The optimum activities of SGC digestive enzymes were detected at pH range of 5.0–6.0 which were almost in accordance with pH value of SGC tissue [7]. This congruence reveals that condition of SGC maintains an optimum environment for salivary digestive enzymes. The accordance of the SGC pH value with the optimum pH for enzyme activities (α-amylase and glucosidases) was reported in other phytophagous bugs. Optimum activities of amylase and proteases in salivary glands of giant waterbugs, Lethocerus uhleri and Belostoma lutarium, were determined to occur at pH of 7.5 [11]. This difference in the pH value for optimal enzymes activity could arise from a difference in feeding habitats, since E. integriceps is a phytophagous bug, while L. uhleri and B. lutarium are predatory bugs.
Salivary α-amylase showed activity against starch as well as glycogen. The presence of α-amylase activity in the SGC was expected, since this insect lives on wheat grains, which is a carbohydrate-rich diet. The activity of the α-amylase against starch, however, was higher than that of glycogen, which shows that this enzyme is developed to digest plant materials, especially wheat seeds. It has been suggested that phytophagous bugs usually have high amylolytic activity in their salivary glands, i.e. plant-feeding mirids [14,25–28], and pod-sucking bugs [13]. In addition, α-amylase has also been reported to be present in salivary glands of the predatory, or partially predatory terrestrial Heteroptera Geocoris punctipes, Orius insidiosus [29,30], L. uhleri and B. lutarium [11], suggesting that these bugs have the ability to digest starch and probably glycogen to obtain nutrients from different feeding sources. The secreted amylase is thought to be ingested through the food canal with partially digested starch and to pass into the alimentary canal, where it is further digested by digestive enzymes and absorbed [4,5].
Fractionation of SGC α-amylases revealed that various fractions contained different isoamylases with different biochemical characteristics. It can be hypothesized that this insect produces different isoamylases with different properties to feed on different food sources. Considering the mode of feeding in this insect, e.g. pre-oral digestion, having a number of isoamylases in salivary secretion would guarantee efficient hydrolyzing action of the enzyme because a group of amylolytic enzymes with different properties is injected to microenvironments (where saliva injected and need to be active) with unpredicted characteristics, e.g. pH, enzyme inhibitors, etc. It has also been reported that insect pests have more than one α-amylase isozyme excreted by digestive tissues. The presence of a number of α-amylases isozymes is a strategy to escape inhibitor toxicity [7,31].
After deriving to small oligosaccharides by the hydrolyzing action of α-amylases on polysaccharides, they are then digested by glycosidases like α- and β-glucosidases and galactosidases. Amylase and glucosidases are indicative of phytophagy [25] and plant-feeding bugs usually have high levels of these enzymes in their salivary gland complex [26]. Revealing its important function in pre-oral digestion in E. integriceps, α-glucosidase showed the highest specific activity among the glycosidases assayed. Glucosidases (α/β) catalyze the hydrolysis of terminal, non-reducing α- and β-1,4 linked glucose residues from arylglucosides such as disaccharides or oligosaccharides. The presence of glucosidases in salivary glands of other phytophagous has also been reported [13,27]. Glucosidase activities in predatory bugs were found to be absent or to be low [4,26,27]. This discrepancy might arise from different source of feeding of predatory bugs with phytophagous ones.
In addition to have a role in digestion, β-glycosidases are important in insect–plant relationships. Plants synthesize a large number of toxic glucosides to avoid herbivores [32]. Almost all plant glycosides are β-linked o-glycosyl compounds, which make them suitable to hydrolysis by β-glycosidases. Thus, aglycones are liberated by the action of β-glucosidase. However, they are usually more toxic than the glycosides themselves. Then, the hydrolyzing activity of β-glucosidase against plant glycoside is detrimental to phytophagous insects. Therefore, it is possible that the cause of a low level of β-glucosidase activity in the SGC of the sunn pest could be to avoid intoxication by plant glucosides. This kind of hydrolyzing avoidance has been reported for other phytophagous insects [33], where Diathraea saccharalis larvae decreased the activity of β-glycosidases in their midgut after feeding on diets containing the cyanogenic glucoside amygdalin. Hydroxamic acids, for example, have been identified as resistance factors against insect pest [34]. Therefore, the pest expresses low levels of β-glucosidase in its saliva to avoid intoxication by plant glucosides. α-d-Galactosidases (EC 3.2.1.22) catalyze the hydrolysis of α-d-galactosidic linkages in the non-reducing end of oligosaccharides, galactomannans, and galactolipids [35]. β-d-Galactosidase (EC 3.2.1.23) is a hydrolase enzyme that catalyzes the hydrolysis of β-galactosides into monosaccharides. With respect to presence of diverse oligosaccharides in the wheat grains, the presence of glalactosidases in the SGC of the insect was expected. Both α- and β-galactosides were present in SGC extracts. The activity of α-galactosides has been reported in another phytophagous bug, Dysdercus peruvianus, with an optimum pH of 5.0 [36].
α-/β-Mannosidases catalyze the hydrolysis of terminal, non-reducing α- and β-d-mannose residues in α- and β-d-mannosides, respectively. It seems that mannosidase activity has generally a low level of activity in the salivary secretion of true bugs, since there is no obvious report of the activity of this enzyme in salivary secretion of bugs, which is true for the Sunn pest, too. Low level of activity might have made it hard to detect this enzyme in salivary glands. An activity of this enzyme has been reported from the midgut of D. peruvianus; however, this activity derived from the meal [36].
Phosphate moieties need to be removed from phosphorylated compounds prior to absorption. This is accomplished by non-specific phosphatases. Based on the activity of the phosphatases in an alkaline or acid medium, they are classified as alkaline phosphatase (EC 3.1.3.1) or acid phosphates (EC 3.1.3.2) [37]. Our result showed that the major phosphatase activity was achieved in acid medium. These results are completely in accordance with those reported for D. peruvianus [36]. In D. peruvianus, both acid and alkaline phosphatase activities were detected in salivary glands; however, acid phosphatase had a significantly higher specific activity than alkaline phosphatase. Acid phosphatase activity in salivary glands of several hematophagous bugs, e.g. Reduviidae and Triatominae, has also been reported [38]. Taken together, it can be concluded that true bugs generally rely on their acid phosphatase to remove phosphate from phosphorylated compounds.
Storage lipids in seeds are triacylglycerols (triglycerides) and they are hydrolyzed by lipases. Triacylglycerol lipases (EC 3.1.1.3) are enzymes that preferentially hydrolyze the outer links of triacylglycerols [37]. We did not find any lipase activity in the SGC extract of E. integriceps using PNpB as a substrate. It has been suggested that digestive lipase is an enzyme specific for zoophagy [6]. However, it should be noted that we detected some lipase activity in the midgut of E. integriceps, suggesting that lipid digestion occurs in midgut rather than at the pre-oral stage (unpublished data).
We detected a serine protease activity in the salivary glands of E. integriceps, which was induced in response to a feeding pulse. The presence of different serine protease activities in this insect was also reported [39]. A serin protease was also purified from wheat seeds damaged by E. integriceps with specificity against glutenin, the major protein within wheat flour [40]. Together, these findings show the specific digestive function of the salivary serine proteases of this insect.
5 Conclusion
In conclusion, it is showed that the SGC of E. integriceps has a typical morphology of heteropteran SGC. This insect produced several digestive enzymes (e.g., α-amylase, α/β-glucosidases, α/β-galactosidases, α/β-mannosidases, and alkaline/acid phosphatases) in its SGC, which is in accordance with the high carbohydrate content in wheat that needs to be digested by the insect. The activities of all detected enzymes were increased in response to feeding, revealing that their production was induced by the feeding pulse. This insect produced several isoamylases in the SGC, indicating its importance in the insect's feeding. The information presented here gives a more detailed insight into the digestion physiology of this insect, especially into pre-oral digestion. Moreover, the identification of saliva biochemical components allows us to get a clearer understanding of insect–plant interactions and of the ecophysiology of feeding and digestion in insects.
Acknowledgement
The authors would like to acknowledge the Iran National Science Foundation (INSF) for their financial support (grant No. 86025.11).