1 Introduction
Hypericin is a red pigmented aromatic polycyclic naphthodianthrone with unique structural and functional properties, isolated from certain species of the genus Hypericum (family Clusiaceae). The genus comprises 484 species under 36 sections distributed all over the world either naturally or as introduced species [1]. Interestingly, hypericin is detected only in species belonging to phylogenetically advanced sections like Hypericum (Hypericum perforatum, Hypericum maculatum), Adenosepalum (Hypericum montanum) and Drosocarpicum (Hypericum umbellatum, Hypericum boissieri), and absent in primitive sections [1,2]. However, recently it was found that Hypericum hookerianum, sparsely distributed in the forests of Western Ghats region in peninsular India, is yet another source of hypericin. Furthermore, the in vitro shoot cultures are amenable for enhanced production of total hypericins by PGR [3] and light induction [4].
Despite being a potent photosensitizer in photodynamic therapy (PDT), many such activities as antidepressant, antiviral, antiretroviral, antineoplastic and antitumor have been attributed to hypericin, which has direct or indirect applications in the clinical and pharmaceutical arena [5–11].
Hypericin being a distant analog of para-quinone, its fundamental structure is similar to that of anthraquinones, as shown in Fig. 1A. However, compared to anthraquinones, hypericin has a much more extensive aromatic system wherein the three rings have been replaced by an eight-ring structure. Hypericin in pure form was first isolated by Brockmann [12], who proposed an initial structure which was later modified into the present structure, as shown in Fig. 1B. Though chemical synthesis of hypericin from emodin anthrone resulting in ca 63% yield of protohypericin is possible [13], further scaled-up production is not cost effective. Evidently, literature on hypericin is largely focused on the influence of biotic and abiotic factors on hypericin production in the field plants and in vitro culture systems [14–16], while reports on metabolic pathways leading to in vivo synthesis of the compound are rather scanty. The biogenesis and evolution of pathways, leading to the synthesis of hypericin, is only hypothesized, but not scientifically validated and hence remains poorly understood [17,18]. As hypericin belongs to the broad category of anthroquinones, the prevailing hypothesis is that hypericin biosynthesis must be mediated through polyketide pathway [19]. It is presumed that biosynthesis of hypericin is likely to start with the condensation of one molecule of acetyl CoA with seven molecules of malonyl CoA to form a linear octaketide chain. This subsequently undergoes specific cyclization to form emodin anthrone, believed to be the immediate precursor of hypericin, catalyzed by a functionally divergent PKS [20,21]. However, till date, no such PKSs showing in vitro activity of converting acetylated substrate to the expected tri/octaketide product (emodin/hypericin) through a condensation and cyclization reaction has been reported in any hypericin-producing species of Hypericum. Although two cDNAs encoding polyketide synthases designated as HpPKS1 and HpPKS2 were isolated from H. perforatum [22], the recombinant protein failed to convert the acetylated substrates to emodin or hypericin under in vitro conditions [23].

A (Color online.) The three-carbon ringed structural unit of anthraquinones showing characteristic numbering of rings where substitution of chemical groups occur (B). Chemical structure of hypericin showing the 8-carbon ring structure flanked by methyl and hydroxyl groups at positions C-10, 11 and C-3,4 respectively.
The isolation and characterization of yet another gene termed hyp-1, proposed to be catalytic in later steps of hypericin synthesis, precisely the conversion of emodin dianthrone to hypericin [24], was later found to be negatively correlated with the hypericin content in different tissues of the plant [25]. Subsequent research established that hyp-1 is not a limiting factor for hypericin biosynthesis in Hypericum [26]. Recent report reveals that hyp-1 is a typical PR-10 family member, inducible by biotic or abiotic stress factors, involved in the plant-defence mechanism [17]. The hypothetical PKS pathway and putative role of hyp-1 gene, along with chemical routes to hypericin synthesis, are schematically shown in Fig. 2A and B.
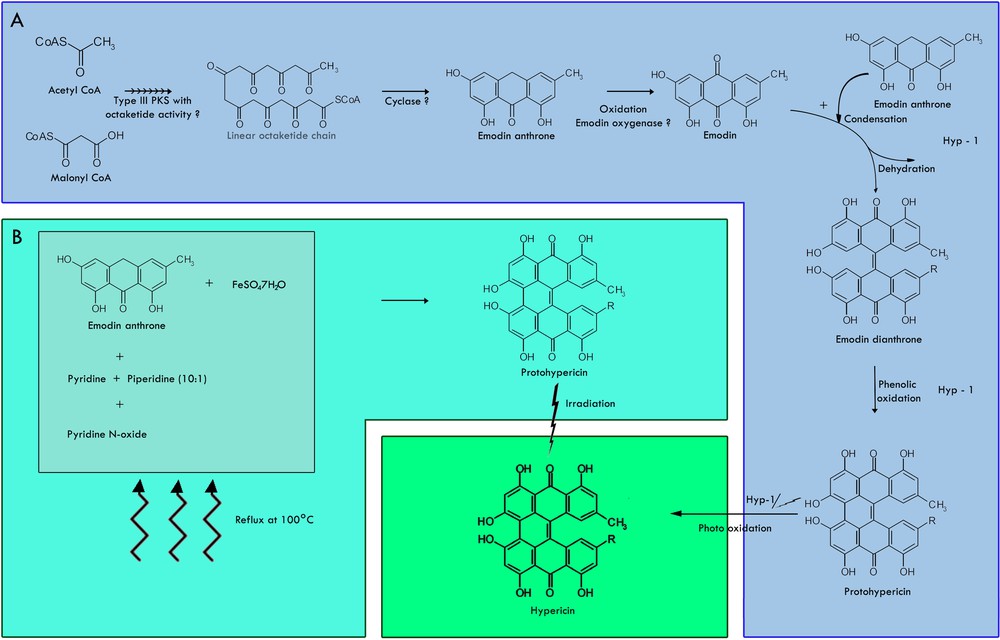
(Color online.) Schematic representation of hypericin biosynthesis by (A) the proposed PKS pathway initiated by an unidentified type-III PKS with possible involvement of a cyclase and an oxygenase followed by repeated steps of condensation/decarboxylation and phenolic/photo oxidations, all catalyzed by a single hyp-1 enzyme, (B) chemical synthesis of hypericin with precursor emodin anthrone in a solution of pyridine and piperidine in the presence of FeSO4·7 H2O.
It is therefore pertinent to revisit the hypothesis, to include/exclude other alternative routes if any, and establish experimentally the existence of PKS-mediated biosynthesis of hypericin. As the basic structural unit of hypericin is emodin anthrone, our search for possible biosynthetic routes for anthraquinone (AQ) synthesis in higher plants revealed the existence of an alternative pathway, viz., chorismate/o-succinylbenzoic acid pathway [27], in addition to the well-characterized polyketide pathway. While some plant families like Leguminosae, Rhamnaceae and Polygonaceae predominantly favor polyketide pathway for anthraquinone biosynthesis [28,29], others, like Rubiaceae and Gesneriaceae, operate the chorismate/o-succinylbenzoic pathway for the synthesis of quinones. Here, the rings A and B (Fig. 1A) are biosynthetically derived from chorismic acid and α-ketoglutarate via o-succinylbenzoic acid (OSB), while ring C is formed via terpenoid pathway. The next level of complication is that terpenoid biosynthesis per se exists through two different pathways, viz., the classical mevalonic acid pathway (MVA pathway) and the recently discovered platidial MEP (methylerythritol phosphate) pathway. The overall schematic representation of pathways, leading to anthraquinone biosynthesis through the alternative pathway, is shown in Fig. 3. As AQ synthesis in Hypericum could follow any of these pathways, it is essential to establish the one that is operational in the plant by excluding the other.

(Color online.) Schematic representation of the alternate pathway comprising MVA, MEP and Shikimate leading to the biosynthesis of anthraquinones in higher plant families. The specific sites of inhibition by the metabolite inhibitors are shown in red lines. DHNA, 1,4-dihydroxy-2-naphthoic acid; DMAPP-3,3, dimethylallyl diphosphate; E-4-P, erythrose 4-phosphate; HMG CoA, 3-hydroxy-3-methylglutaryl coenzyme A; HMGR, 3-hydorxy-3-methylglutaryl coenzymeA reductase; IPP, isopentenyl diphosphate; MVA, mevalonic acid; MEP, 2-C-methyl-d-erythritol 4-phosphate; OSB-o-succinylbenzoic acid; TPP, thiamine diphoshate. Enzymes: ics, isochorismate synthase; OSBS, o-succinylbenzoate synthase; DXR, 1-deoxy-d-xylulose 5-phosphate reductoisomerase; DXS, 1-deoxy-d-xylulose 5-phosphate synthase.
We used metabolite inhibitor studies for possible inclusion/exclusion of the alternate pathway in emodin synthesis, as inhibitors for functionally divergent PKSs are not available. The compelling reasons to consider alternate pathway in emodin synthesis are briefly discussed here:
- • Presence of emodin across plant families.
Emodin, chemically known as 6-methyl-1,3,8-trihydroxyanthraquinone, is not taxonomically restricted to Hypericum, but occurs in at least 94 plant species across 28 genera of 17 plant families [30], which do not produce hypericin. Furthermore, PKSs with triketide synthase activity leading to the production of emodin have not been reported from any of these plants.
- • Substitution pattern in emodin.
The AQs derived via the polyketide pathway often exhibit a characteristic substitution pattern, i.e. they are substituted in both rings A and C (Fig. 1A) of the AQ structure. Emodin, with typical substitution at position C8 and C3 of the rings A and C respectively, with hydroxyl groups in fact, claims a polyketide origin. Interestingly, many of the quinones derived via polyketide pathway, have this kind of substitution due to a characteristic oxygenation pattern called metaoxygenation, taking place during the ring formation. However, there are many plant-derived AQs like alizarin, morindone and others in the family Rubicaeae showing exactly similar substitutions of hydroxyl, methoxy and methyl groups in both the ring A and C as well, but are derived via the alternate pathway and not from polyketide pathway [27]. Therefore, it is not possible to ascertain the biosynthetic origin of emodin based on substitutions in rings A and C.
- • Influence of PGR in AQ production.
The majority of the AQs, reported to have been either induced or suppressed by auxin action, are all derived from shikimate pathway. In our earlier study also [3], we have found that the synthetic auxin NAA at 0.2 mg L−1 (shoot cultures) or 2.0 mg L−1 (callus cultures) enhanced hypericin production to manifold. Therefore, it is reasonable to assume that AQ production could be mediated through the alternate pathway in H. hookerianum.
In the present investigation, we have used specific metabolite inhibitors to block the biochemical pathways and SSH analysis, for differential expression of genes related to hypericin synthesis, under control and elicited conditions. To the best of our knowledge, the strategy was not reported in any of the Hypericum spp., till date.
2 Materials and methods
2.1 Plant material and culture condition
Collection of plant materials, development of in vitro culture systems, establishment of high yielding shoot and shoot-forming callus cultures using 0.2 and 2.0 mg L−1 NAA, respectively, and optimized culture conditions are as described earlier [3]. The voucher samples after confirming their taxonomic identity were deposited in the JNTBGRI herbarium under number TBGT-46936.
2.2 Metabolic pathway inhibition using specific inhibitors
Stock solution of all the three metabolic pathway inhibitors, viz., mevinolin, fosmidomycin and glyphosate were prepared, membrane filtered and added to MS nutrient formulation having full-strength salts, vitamins, 3% sucrose and supplemented with 1.0 mg L−1 Kin and 0.20 mg L−1 NAA (0.15% phytagel pH 5.8), at varying concentrations ranging from 1 to 50 μM (fosmidomycin and glyphosate) and 10 to 500 μM (mevinolin). Culture medium without NAA served the uninduced control system. Shoot clumps from stock cultures, maintained in same medium, were subcultured in media containing varying concentrations of these metabolite inhibitors. Total hypericins were extracted after four weeks. Control cultures were cultivated in the same medium, but without inhibitors. However, all experiments had auxin-induced and control cultures to study the effect of the inhibitor on both callus and shoot cultures.
2.3 Extraction and quantification of hypericins from shoot cultures
100 g fresh weight (FW) of red pigmented fresh shoots induced by auxin treatment and control green shoot cultures were harvested and extracted in a soxhlet apparatus for 3–4 h with 50 mL of diethyl ether, to remove chlorophyll pigments. The residue was dried and re-extracted in a soxhlet apparatus for another 6–8 h with 50 mL of ethyl alcohol. The extract was then filtered, dried and the residue resuspended in 5.0 mL of methanol for HPLC estimation. As the callus is devoid of chlorophyll, the extraction was done only with ethyl alcohol, keeping other parameters identical.
High-performance liquid chromatography (HPLC) was carried out for the estimation of hypericins in these culture systems following the standard procedure [31] with appropriate modifications, as reported earlier [4]. In brief, Reverse Phase column (C18, 125 × 4 mm) of the HPLC system (Gilson, USA) was equilibrated with solvent systems comprised of (A) acetonitrile: (B) methanol and water (70:30) in the ratio (A:B) 20:80. An aliquot of 25 μL of each sample was injected into the column and the flow rate was maintained at 1 mL min−1. The column temperature was set at 20 °C. The elution was monitored at 590 nm and the data obtained were compared with those of authentic hypericin (Sigma-Aldrich, USA). The estimation of hypericin in the individual samples was calculated from the calibration curve prepared in the range from 0.5 to 100 μg mL−1 (r2 = 0.999). Each sample was analyzed thrice and the mean value was taken for calculation.
2.4 Differential screening of auxin-induced and control shoot cultures using SSH
2.4.1 mRNA isolation from in vitro shoot cultures
Total RNA from driver (control shoot cultures without NAA supplementation) and tester (shoot cultures in medium supplemented with 2.0 mg L−1 NAA) was extracted following the protocol [32] using guanidium isothiocyanate. The final pellet was resuspended in 40 μL of DEPC treated autoclaved water and stored at–70 °C. From total RNA, mRNA was isolated by Polytract mRNA isolation system III (Promega Corporation, USA) following the manufacturer's protocol. Since a high concentration of mRNA (2 μg in 1–4 μL) is required for first strand synthesis, the eluted sample in 100 μL was enriched with mRNA by adding 20 g glycogen, 0.1 volume 3 M sodium acetate buffer (pH 5.2) and 3 volumes of ice-cold absolute alcohol. The final pellet was re-suspended in 10 μL RNAase free water. The concentrations of both total RNA and mRNA were determined spectrophotometrically using an Eppendorf BioPhotometer (Germany). As callus cultures could not yield the desired amount and purity of RNA, differential screening was continued only with shoot cultures.
2.4.2 cDNA library construction by Suppression Subtractive Hybridization
Subtracted library was constructed by using the PCR-Select cDNA subtraction kit (Clonetech, USA) following the instruction manual. The subtracted products, after a first and a second hybridization, were PCR-amplified to normalize and enrich differentially expressed cDNAs, followed by selective amplification by nested PCR, to further reduce the background. The subtracted products were fractionated on a 1.8% agarose gel. The forward subtracted products were eluted using GenElute gel extraction kit (Sigma-Aldrich, USA). The eluted products were ligated to pGEM-T Easy vector after determining the appropriate vector: insert ratio.
2.4.3 Analysis of the subtracted library
The transformed positive clones were selected following a blue/white screening method and plasmid isolated using the plasmid isolation kit (Sigma-Aldrich, USA). Inserts from the recombinant plasmids were amplified using T3 and T7 sequencing primers. The PCR reaction was carried out in a 20-μL volume containing 15 ng of plasmid DNA. Thermal cycling conditions were: 94 °C for 3 minutes followed by 25 cycles of 94 °C for 30 s, 55 °C for 30 s and 72 °C for 1 min.
2.5 Heterogeneity check of the recombinant clones
Restriction analysis was carried out in 10 μL of the PCR product using enzymes with 4-bp (RsaI and TaqI) or 5-bp (HinfI) recognition sequences. To the digest, 4 μL of 6X loading dye were added and fractionated on 2% agarose gel along with suitable molecular-size standards. Clones were grouped based on their restriction pattern.
2.6 DNA sequencing and homology search
DNA sequencing of the differentially expressed clones were carried out using the 3130 Genetic Analyzer (Applied Biosystems, USA) facility available in our laboratory. Homology searches were performed with BLAST [33] and BLASTX [34] algorithm against the non-redundant version of the sequence databases at the National Centre for Biotechnology Information (NCBI).
3 Chemicals
All molecular biology grade reagents for DNA, RNA, plasmid isolation and gel electrophoresis along with metabolite inhibitors mevinolin and glyphosate and hypericin standard were purchased from Sigma-Aldrich, USA. Fosmidomycin was purchased from Life Technologies. SSH kit was purchased from Clonetech, USA and pGEM-T Easy vector from Promega Corporation, USA.
4 Results
4.1 Effect of metabolite inhibitors on alternate pathway of hypericin synthesis
Metabolite inhibitors, which are known to block specific regulatory steps of the alternate pathway of AQ synthesis, were used in the present investigation (Fig. 3). The effect of varying concentrations of the inhibitors, ranging from 1 to 50 μM (glyphosate and fosmidomycin) and 10 to 500 μM (mevinolin) on hypericin synthesis, in 30-day-old control and auxin-induced shoot and shoot-forming callus cultures of H. hookerianum, were analyzed and estimated using HPLC (Fig. 1S). Although, all three inhibitors at higher concentration were detrimental to cell growth, as they did not inhibit hypericin synthesis at any significant levels. Mevinolin, the inhibitor of HMGR rather induced hypericin synthesis at initial concentrations of 10 to 100 μM in the cultures (Fig. 4A), resulting in average synthesis of 2.80 ± 0.22 and 18.75 ± 0.01 mg g−1 DW in shoot and 16.39 ± 3.75, 29.60 ± 1.90 mg g−1 DW hypericin in callus, under control and induced conditions of growth, respectively. Similarly, fosmidomycin another inhibitor of the terpenoid pathway, though showed transient decrease at the initial concentration (1 μM) but increased the levels of hypericin in the callus cultures at concentrations 5 to 25 μM, resulting in average of 2.53 ± 0.10, 18.12 ± 0.56 mg g−1 DW in the shoot cultures and 0.14 ± 0.01and 14.28 ± 1.11 mg g−1 DW hypericin in the callus cultures, under control and induced conditions (Fig. 4B). Glyphosate, the inhibitor of the shikimate pathway responsible for the synthesis of ring A & B, also showed elevated levels of hypericins from 7.5 to 14.6 mg g−1 DW at inhibitor concentrations from 1 to 10 μM in induced calli, while induced shoot cultures showed higher total hypericins (21 mg g−1 DW) at 5 μM followed by gradual decrease to 16.8 mg g−1 DW, with an incremental increase of glyphosate concentration (Fig. 4 C). The decrease in biomass yield, at higher inhibitor concentration, showed a corresponding decrease in hypericin production.

(Color online.) Effect of varying concentrations of metabolite inhibitors. (A) mevinolin, (B) fosmidomycin, and (C) glyphosate in hypericin synthesis and biomass production in the auxin-induced and control shoot and shoot-forming callus cultures of Hypericum hookerianmum.
Biomass production in terms of fresh weight showed declination, if not drastically, at higher concentration of all inhibitors, irrespective of the pathway arrested. However, the biomass yield of undifferentiated callus cultures exhibited slightly different patterns in their response to metabolite inhibition. For instance, control calli (without auxin supplementation) at both fosmidomycin and glyphosate inhibition, showed an increase in biomass yield ranging from 3.9 to 5.8 and 3.8 to 6.1 g FW respectively, with an incremental increase of inhibitor concentration. However, induced calli showed reduced biomass under both treatments. The shoot cultures, which were green initially, turned slight reddish at the base, which was in close proximity with the medium and gradually turned brown with shoots losing their robustness with a dosage increase of the metabolite inhibitors. Unlike callus cultures, decline in biomass yield in shoot cultures was uniform and followed a regular pattern, wherein control cultures showed relatively a steep decrease in biomass (26.5 to 6.75, 27.1 to 6.1 and 26.2 to 5.89 g FW), as against induced cultures (29.18 to 17.07, 28.40 to 16.30 and 28.14 to 16.90 g FW) with mevinolin, fosmidomycin and glyphosate concentrations, respectively.
4.2 Differential expression of sequences in shoot cultures under auxin-induced and control conditions
Hypericin-rich shoot cultures, obtained by in vitro manipulation of the culture media as reported earlier [3], were taken as the test sample and shoot cultures in basal MS without NAA supplementation were taken as the driver. To ensure the efficiency of the whole experiment, seven independent PCR reactions were carried out with sufficient control reactions. The subtracted products of the forward reaction, when separated on 1.8% agarose gel, showed five major amplicons of size ranges from 300 to 650 bp (Fig. 5). These amplicons, upon sub-cloning using pGEM-T easy vector and subsequent blue/white screening, resulted in 180 positive clones. To further confirm the heterogeneity of the isolated clones, restriction digestion of the samples were carried out with four-base (RsaI and TaqI) or five-base pair (HinfI) recognizing restriction enzymes. The products were fractionated and restriction patterns compared, between the clones derived from a single product. The sum of the molecular weights of all the restriction fragments, as observed, was greater than the molecular weight of the original product, thus indicating its heterogeneity (Fig. 2S). Following the analysis, the 180 clones isolated were separated into 25 restriction groups. A minimum of one clone from each of the 25 restriction groups identified, amounting to a total of 142 clones, were sequenced. Homology searches using BLASTX and BLASTP algorithm indicated that 125 out of the 142 clones are indeed homologous to different sets of known and unknown proteins. It is observed that the level of identity of cloned sequences to known proteins ranged from 28 to 81% and 38 to 91% with unknown/hypothetical proteins. Functional annotation of these sequences showed that 38% of them belong to protein synthesis, 20% on modification and 9% on electron transport to various metabolic pathways, while the remaining ones are comprised of unclassified (11%) and unknown/hypothetical proteins (22%). The data is represented in the form of a pie chart (Fig. 6). Interestingly, there are sequences showing homology to enzymes involved in secondary metabolism in plants, like hydroxycinnamoyl transferase, monodehydroascorbate reductase and specifically to polyketide synthase, besides several modifying enzymes. A representative set of sequences deposited in the NCBI database is available under the accessions numbers EE665978 to EE665992.

SSH profile of hypericin rich (auxin-induced) and hypericin low (control) producing in vitro shoot cultures of H. hookerianum following forward and reverse subtraction. M: 100 bp DNA ladder (NEB). Lane 1: subtracted control from kit. Lane 2: subtracted skeletal muscle cDNA. Lane 3: unsubtracted skeletal muscle cDNA. Lane 4: forward subtracted cDNA resulting in five specific amplicons. Lane 5: forward unsubtracted cDNA. Lane 6: Reverse subtracted cDNA. Lane 7: reverse unsubtracted cDNA.

(Color online.) Functional annotation of differentially expressed sequences obtained from SSH data showing the percentage of non-redundant sequences grouped as sequences of unknown function and sequences classified into functional groups.
5 Discussion
Despite extensive research on the pharmacological and phytochemical aspects of hypericin, there are only very few reports on the biosynthesis of this compound. The recent report on de novo sequencing of H. perforatum, indicates the screening of many unigenes, and some of them were predicted to be involved in the biosynthesis of hypericins, hyperforin, and melatonin [35]. Although many are annotated, they include genes for both polyketide and MEP pathways and as such do not provide conclusive information on the veracity of the pathways specific to hypericin synthesis. Though there are few PKSs like BPS and CHS from H. androseamum [36] and functionally divergent non-CHS type PKS1 and 2 from H. perforatum [22,23] isolated, they are not involved in hypericin biosynthesis to any significant extent, as demonstrated by in vitro experiments using the recombinant enzyme.
Emodin, the penultimate precursor of hypericin being an anthrone, we shifted our focus on AQ biosynthetic pathway, as it will be shared to a larger extent for hypericin synthesis except for the downstream steps. As AQ synthesis in plants is operated by polyketide synthase (PKS) and chorismate/o-succinylbenzoic acid (alternate) pathways respectively, we used metabolite inhibitor studies for possible inclusion/exclusion of the alternate pathway in emodin synthesis, as inhibitors for functionally divergent PKSs are not available.
5.1 Exclusion of alternate pathway in hypericin biosynthesis by inhibitor studies
The inhibitors used in the present investigation, are known to block specific steps in the secondary metabolic pathway of plants. Glyphosate (N-[phosphonomethyl] glycine), a potent broad spectrum herbicide widely used in agriculture [37] is also a specific inhibitor of the shikimate pathway, while mevinolin, a fungal metabolite isolated from the cultures of Aspergillus terreus, is a potent competitive inhibitor of 3-hydroxy-3-methylglutaryl-coenzyme A reductase of the classical mevalonate pathway [38], fosmidomycin (3[formyl(hydroxy)amino]propylphosphonic acid) is an antibiotic that specifically inhibits DXP reductoisomerase, a key enzyme in the MEP pathway of isoprenoid biosynthesis [39]. Different concentrations of glyphosate ranging from 1 to 50 μM, when applied to shoot and shoot-forming callus cultures of H. hookerianum, did not show any decrease in hypericin production after four weeks, showing that glyphosate did not inhibit hypericin production, but arrested cell growth. The inhibitors of terpenoid pathway, viz., mevinolin and fosmidomycin, also arrested cell growth at higher concentrations, but not hypericin production. The slight increase in hypericin production, at 10 μM and 50 μM concentrations of mevinolin, may be the result of stress-induced production of the defense molecule, but failed to do so at higher concentration of the inhibitor, as the cell growth was adversely affected at high concentrations (100 to 500 μM). As tissue growth and product formation are mostly observed to be inversely related in plant tissue culture, the observed deterioration of cell growth at higher inhibitor concentration could be partially attributed to disruption of primary metabolism due to enhanced secondary metabolite production and also to other lethal effects of the inhibitor to growth and differentiation of the cell. Somewhat normal but distorted anatomy of cells, in the sub-epidermal cortical region of the stem and abnormal changes of cells in the leaves, were noted in shoot cultures of H. hookerianum, due to overproduction of hypericins [4].
Previous workers have reported effective inhibition of the target sites by these metabolic inhibitors as glyphosate, inhibitor of the shikimic acid pathway, has been shown to inhibit AQ production in cell cultures of Galium mollugo. However, this inhibition can be alleviated by the addition of chorismate or OSB, apparently indicating that glyphosate inhibits the step from shikimic to chorismic acid [40]. Similarly, glyphosate decreased AQ production in cell cultures of Cinchona ledgeriana and this inhibition was transcended by the addition of l-tyrosine, l-phenylalanine and l-tryptophan [41]. The effect of terpenoid inhibitors in the production of shikonin by cell suspension cultures of Arnebia euchroma is that higher concentrations of mevinolin inhibited the production of shikonin by 93%, whereas fosmidomycin arrested the production by 50% [42]. Similarly, mevinolin inhibited shikonin biosynthesis in Lithospermum erythrorhizon by 98%, indicating the species preference to MVA pathway over MEP for terpenoid synthesis [43]. Therefore, the metabolite inhibitors used in the present study are indeed potent chemicals capable of blocking ring formation at specific steps in the respective pathways and thereby inhibiting the synthesis of emodin provided; the synthesis is mediated by the alternate pathway. However, the continuous production of hypericins even at higher concentrations of inhibitors indicates regular supply of emodin precursor to hypericin biosynthesis, which unambiguously is mediated by PKS pathway. The only other inhibitor study reported in Hypericum, using PAL inhibitor (phenylalanine ammonia-lyase), showed decreased production of hypericin in the shoot cultures of H. perforatum and H. canariense [44]. However, PAL being a pivotal enzyme in general phenylpropanoid pathway, the observed decrease in hypericin production could not be related to presumptive step(s) committed to hypericin production.
5.2 SSH analysis to detect differentially expressed sequences under auxin-induced and control conditions
Differential screening of auxin-induced and control shoot cultures of H. hookerianum (Fig. 3S) using SSH resulted in 142 differentially expressed sequences. Functional annotation of these differentially expressed sequences showed that 67% of them are involved in secondary metabolite synthesis, in one way or another. The significance of these sequences are highlighted, particularly in the context where many type-III plant PKSs have recently been found to be incapable of performing expected in vivo reactions under in vitro experimental conditions. Although the enzyme accepts the suggested starter substrate and performs the correct number of condensations with malonyl CoA, it fails to cyclize the linear polyketide chain to the predicted final products [45–49]. The classical example is the production of shunt products SEK4 and SEK4b by OKS in Aloe arborescens and HpPKS2 in H. perforatum, respectively. Here, instead of the expected octaketides, due to lack or improper cyclization of linear octaketide chain [20], shunt products are formed. This is attributed to the absence of tailoring enzymes like cyclase-like cofactor or reductase in the reaction mixture. These additional protein partners are assumed to stabilize the highly reactive linear polyketide chain, preventing non-specific cyclizations to occur [50,51]. The presence of sequences, showing similarity to monoascorbate reductases, acetyl transferases, and hydrogenases in the present study confirms the necessity of tailoring enzymes, for effective cyclization of emodin anthrone to form the octaketide hypericin. It is further presumed, that a transporter protein may be involved in hypericin biosynthesis, so as to facilitate the long distance transport of hyp-1 protein, supposed to be responsible for the conversion of emodin anthrone to hypericin [21]. The differentially expressed sequences, isolated in the present study, indicate their possible role in hypericin biosynthesis; however, their actual involvement as tailoring enzymes or transfer proteins or PKS with functional divergence belonging to non-CHS class entails further experimental evidence.
In conclusion, the results of the current study provided, for the first time, direct biochemical and molecular evidences for PKS-mediated synthesis of hypericin in Hypericum, which otherwise remained a hypothesis with little experimental validation. The results obtained provide valuable insights into hypericin biosynthesis and form basis for further investigation.
Disclosure of interest
The authors declare that they have no conflicts of interest concerning this article.
Acknowledgments
This work was supported by a research grant from the Department of Biotechnology, Govt. of India (No. BT/PR/4023/AGR/16/334/03). We greatly acknowledge the Directors of JNTBGRI and NIT-C for extending all facilities required for the conduct of relevant experiments. We are also thankful to the Forest Department, Govt. of Tamil Nadu for permitting collection of plant samples from the Nilgiris forest region.
Contribution of authors: P.P. conceptualized the research problem, conducted metabolite inhibitor and SSH studies and R.A.N. conducted all cloning experiments and statistical analysis of data. Both authors prepared the manuscript together.