1 Introduction
Cadmium has become a common pollutant in aquatic environments, with high concentrations often seen in industrialized areas [1]. Once dissolved, it is readily absorbed and bio-accumulated with toxic effects, at all levels of the food chain [2]. In addition, cadmium accumulates in sediments from which it is gradually released with resultant short- and long-term contamination [3].
Exposure to cadmium causes a plethora of negative effects in aquatic organisms. In invertebrates, it generates oxidative stress and time- and dose-dependent deregulation of transcription. For example, it up-regulates the expression of antioxidant enzymes [4], metallothioneins (MTs) and heat shock proteins (HSPs) [5], whereas it down-regulates the expression of digestive enzymes [6], esterases and phospholipase A2 [7]. Cadmium also interferes with immune responses [8,9], tissue organization and histology [10,11], and cell cycles by inducing apoptosis [12].
In teleosts, multiple effects of cadmium contamination have been described for major sites of metal absorption (gills and guts), and in predominant detoxification sites (liver and kidney). In all these organs, the metal causes morphological alterations [13], oxidative stress [14], and induces variations in the expression of several genes (MT, HSP, IGF-I) implicated in stress response [15,16] or in housekeeping activities [17]. Cadmium also decreases growth [18], delays reproduction [19], and reduces swimming performance [20]. Embryo development is markedly affected by cadmium as well. Malformations, increased mortality [21–23], induced oxidative stress [24] and behavioral defects [21] are also reported. Many of these effects are species-specific [25,26].
Cadmium exposure causes significant ocular malformations in fish embryos. Microphthalmia, reduction in the number of retinal ganglion cells, loss of photoreceptors and optic nerve growth failure have been reported. Visual abilities after cadmium exposure are obviously reduced and embryos are behaviorally blind [27]. In contrast, cadmium toxicity in the retina of adult teleosts is poorly known, even though, in these animals, most environmental interactions are based on visual information. For example, prey capture, escape from predators [28], timing of the reproductive cycle [29,30], and camouflage [27] are vision-dependent activities.
This work describes the effects of environmentally realistic concentrations of cadmium on the vision of adult zebrafish (Danio rerio), a preeminent experimental model for vertebrate vision research [31]. Here, we characterize cadmium-induced retinal morpho-cytological alterations by light and electron microscopy and assess visual performance by studying the sensitivity of cadmium-exposed animals to white or colored light by re-illumination tests.
Our results show that cadmium induces significant toxic effects in adult zebrafish with degeneration, loss of retinal organization, and consequent impairment of the functional response, particularly through increased light sensitivity.
2 Materials and methods
2.1 Animal care and treatment
Adult zebrafish (Danio rerio) were acclimatized in 50-L tanks at a temperature of 26 °C with natural photoperiod and fed twice daily. Under our experimental conditions, fish were maintained in 10-L test tanks, placed in a temperature-controlled water bath, aerated and provisioned with a mechanical filter to remove food debris and maintain water circulation. Animals were randomly allotted to three groups and treated as follows: group I received no treatment (control); group II was transferred to water containing CdCl2 at a concentration of 0.3 mg/L (environmental concentration; [32]); group III was transferred into water containing CdCl2 at a concentration of 3.0 mg/L. Treatment was static (solutions remained unchanged throughout the duration of the test) and lasted for 30 days. The experiments were carried out in compliance with ethical provisions established by the EU directive 2010/63/EU for animal experiment and authorized by the National Committee of the Italian Ministry of Health for in vivo experimentation (Dept. for Veterinary Public Health, Nutrition and Food Safety). Animal welfare was maximized by reducing and refining animal use in accord with the 3Rs principle [33].
2.2 Light microscopy
At sampling, animals were anesthetized with MS222 (tricaine methanesulfonate, 1:15000 w/v) and sacrificed by decapitation. Retinae were rapidly dissected, fixed in Bouin's solution and processed for paraffin wax embedding according to routine protocols. Sections were stained with haematoxylin and/or eosin to show general morphology.
2.3 Electron microscopy
For electron microscopy analysis, the retinae were dissected and fixed in 2.5% glutaraldehyde and 4% paraformaldehyde in 0.1 M PBS at pH 7.4 for 24 h at 4 °C, washed in the same buffer and post-fixed in 1% osmium tetroxide in 0.1 M PBS, pH 7.4, at 4 °C for 1 h. They were then dehydrated in ascending series of ethanol and embedded in Epon. Ultra-thin sections were stained with 3% uranyl acetate in 50% ethyl alcohol and with 2.6% lead citrate and observed under a Philips EM 208S transmission electron microscope at 100 kV.
2.4 Behavioral tests
A modification of the behavioral test of Li and Dowling (1997) [34] based on a visually mediated escape response, by dark-adapted adult zebrafish was used in these experiments. The sensitivity to light of different wavelengths was compared in control and cadmium-treated animals.
An undisturbed, environmentally controlled room, with artificial illumination mimicking the natural photoperiod, held test tanks in which single animals were acclimatized for 24 h. The tank, in which the fish could swim freely in a circular motion, consisted of a cylinder (25-cm diameter) containing a smaller (5-cm diameter) opaque cylinder in the middle serving as a light shelter. A small lamp equipped with an aquarium light bulb and a web camera to capture fish behavior responses were mounted above the tank.
At 11 a.m. in the morning following acclimatization, fish were exposed to a 60-min period of dark followed by 1 min of illumination with a white, or red, or yellow, or blue or green light. These were obtained by placing a photographic filter in front of the light bulb. The light was placed very close to the water to create a bright sector in the tank on one side and a relatively “dark” sector, at the opposite side behind the small opaque central cylinder.
The escape response evoked by the light was determined by counting the number of times the fish passed through the bright sector of the tank per minute of observation. Tests at each filtered wavelength were carried out on six animals in each of the three treatment groups. Fifteen untreated animals, exposed to unfiltered diffuse white or colored light were used as blank controls. Results obtained were pooled and examined for significance at P < 0.05 by ANOVA.
2.5 Statistical analysis
The apoptotic cells on TEM sections were quantified on 10 non-overlapping areas of 1080 μm2, considering at least one area in gangliar cell layer and one area in the outer nuclear layer per sample (n). Quantitative data are depicted as mean with standard deviation (SD) obtained from at least three animals tested for multiple comparisons by the one-way analysis of variance (ANOVA) followed by a suitable post hoc t-test; *P < 0.01, **P < 0.001.
3 Results
3.1 Effects of cadmium on retinal morphology
Retinas of control animals were regularly shaped and showed a typical organization with alternated nuclear and fibrous layers (Fig. 1A, B). Functional layering is also visible among the photoreceptors after staining with eosin and exposure to UV light. Rods and the three types of cones are easily distinguishable with their inner and intensely fluorescent outer segments (Fig. 2A).

(Color online.) Morphological alterations in retinae of Danio rerio control (A–B) or exposed to 0.3 (C–E, G) or 3.0 (F, H–I) mg/L cadmium chloride. A. Typical layering: outer (ONL) and inner (INL) nuclear layers, thick inner plexiform layer (IPL), horizontal (arrow) and ganglion (G) cells. Retinal pigmented epithelium (RPE). B. Detail showing the ganglion cell layer (G) and the nerve fiber layer (*). C. Treated retina with altered nerve fiber layer (*). D. Detail showing the presence of pycnotic nuclei (arrow). E and F. Presence of empty areas in photoreceptor layer (arrows) in treated retinae; G. Fold (*). H. Irregular retinal pigmented epithelium (arrow) protruding in the photoreceptor layer. I. Vitreal chamber with blood cells (*). Bars: I: 50 μm; A, C, G, H: 25 μm; B, D–F: 5 μm. A–D, I: Haemalum–eosin staining; E–F: toluidine blue; G: eosin staining, H: no staining.

(Color online.) Changes in photoreceptors in Danio rerio control (A) or exposed to 0.3 (B) or 3.0 (C) mg/L cadmium chloride. A. Regular disposition of the rod nuclei (ONL) and outer segments (*). Cones inner (C) and fluorescent outer (arrow) segments. Retinal pigmented epithelium (RPE). Single short (1), single long (2) and double (3) cones. B. Cone inner segments (C) and the ONL are moderately disorganized. The RPE is thick and dense, the outer segments of rods (*) poorly fluorescent. C. The ONL and the inner segments of cones (C) appear more disorganized. The outer segments of rods (*) is intensely fluorescent. Bars: 10 μm. Eosin staining, observed under UV light.
After treatment with cadmium, dose-dependent alterations with increasing severity at the higher dose are clearly observed. The nerve fiber layer is clearly thickened and vacuolated (Fig. 1C), while several ganglion cells have compact pycnotic nuclei (Fig. 1D). Empty areas are observed, especially in the photoreceptor layer (Fig. 1E, F). In control retinae similar alterations are never observed. The organization of photoreceptors in layers was unaltered but changes occurred in the thickness of the pigmented epithelium and at the level of the cones’ inner segments (Fig. 2B, C). In addition, the treated retinae often exhibit extended folds (Fig. 1G, H). In rare cases, especially at the high dose, the vitreal chamber contains cell debris and/or blood cells (Fig. 1I).
3.2 Effects of cadmium on retinal cell ultrastructure
TEM observations of control retinae reveal correct ultrastructural organization of typical layers (Fig. 3A–D). In fish treated with 0.3 mg/L Cd, retinae confirm the presence of ganglion cells with highly vacuolated cytoplasm (Fig. 3E) and cells with ultrastructural apoptotic features (n = 3: 1.10 ± 0.73; mean ± SD; Fig. 3E, F, Fig. 4). Cilia protruding from the optical layer in the vitreal chamber can also be observed (Fig. 3G). Cells with clear apoptotic features are also evident in the inner nuclear layer (Fig. 3H) and in the photoreceptor layer (n = 3: 1.20 ± 0.92; Fig. 3I, J, Fig. 4). Moreover, Müller cells in the outer nuclear layer are present (Fig. 3I). Most of the cones are in a regular “mosaic” arrangement, but there are some with aberrant horizontal orientation (Fig. 3 K). Pyknotic nuclei of pigmented cells are found among the rod outer segments (Fig. 3L).

Ultrastructural alterations of retinal cells in Danio rerio. Control animals (A-D) or exposed to 0.3 (E–L) or 3.0 (M–P) mg/L cadmium chloride. A. Ganglion cell layer (G) and IPL. B. INL and ONL. C. ONL and cones (C). D. RPE with melanin granules (m) between rods and cones outer segments. E. Apoptotic cells (arrows) among healthy ganglion cell (G). F. Detail of two apoptotic cells with pycnotic nuclei (*). G. Transverse section of a basal body (arrow) and longitudinal section of a ciliary axoneme (arrowhead). H. Inner nuclear layer with apoptotic nuclei (arrows). I. ONL, rods and cones nuclei. J. Apoptotic cells (arrows) among photoreceptors (*). K. Misoriented cone. Notice the cone (arrow) perpendicular to the normal arrangement (*). L. Pigmented cell in apoptosis (arrow) and photoreceptor outer segments (*). M. Significant vacuolation (*) of the ganglion cell layer (G). N. Pycnotic nucleus (arrow) of a ganglion cell. O. INL with polymorphic Müller cells (arrow); P. Rod nuclei with irregular profiles and condensed content in ONL.

Histogram showing the mean of the number of apoptotic cells on 1080 μm2 area, visualized in TEM experiments in controls, or exposed to 0.3 (T 0.3) or 3.0 (T 3) mg/L cadmium chloride, in ganglion cell layer (on the left) and in the outer nuclear layer (on the right). Apoptotic cells/1080 μm2 area in ganglion cell layer (mean ± SD): control (n = 3) 0.00 ± 0.00; T 0.3 (n = 3) 1.10 ± 0.73; T 3 (n = 3) 1.40 ± 0.84. ANOVA P < 0.001; T 0.3 versus control: **P < 0.001; T 3 versus control: **P < 0.001. Apoptotic cells/1080 μm2 area in the outer nuclear layer (mean ± SD): control (n = 3) 0.00 ± 0.00; T 0.3 (n = 3) 1.20 ± 0.92; T 3 (n = 3) 2.40 ± 1.17. ANOVA P < 0.001; T 0.3 versus control: *P < 0.01; T 3 versus control: **P < 0.001.
Similar alterations are found after treatment with 3 mg/L Cd. At the higher Cd exposure concentration, the vacuolation of the optical layer is more severe (Fig. 3M), the apoptotic ganglion cells are more numerous (n = 3: 1.40 ± 0.84; Fig. 3N, Fig. 4) and, in the inner nuclear layer, the number of polymorphic Müller cells is clearly increased (Fig. 3O). In addition, rod nuclei are condensed and have assumed an irregular shape (Fig. 3P). More apoptotic cells are seen particularly in the outer nuclear layer (n = 3: 2.40 ± 1.17; Fig. 4).
The presence of apoptotic cells due to treatment with 0.3 (T 0.3) and 3 (T 3) mg/L cadmium chloride is significant both in the ganglion cell layer (ANOVA P < 0.001; T 0.3 versus control: **P < 0.001; T 3 versus control: **P < 0.001) and in the outer nuclear layer (ANOVA P < 0.001; T 0.3 versus control: *P < 0.01; T 3 versus control: **P < 0.001) (Fig. 4).
3.3 Effects of cadmium on metallothioneins localization
In control retinae, metallothioneins (MT) are present in the inner plexiform layer (Fig. 5A) and in the cones’ inner segments (Fig. 5A–B) that show non-homogeneous protein content (Fig. 5B). MTs are also present in the cytoplasm of the horizontal cells (Fig. 5B). After 0.3 mg/L cadmium exposure, no significant difference in MTs distribution can be observed with respect to control retinae (Fig. 5C). At the higher dose, MTs only increase in the horizontal cells (Fig. 5D and inset).
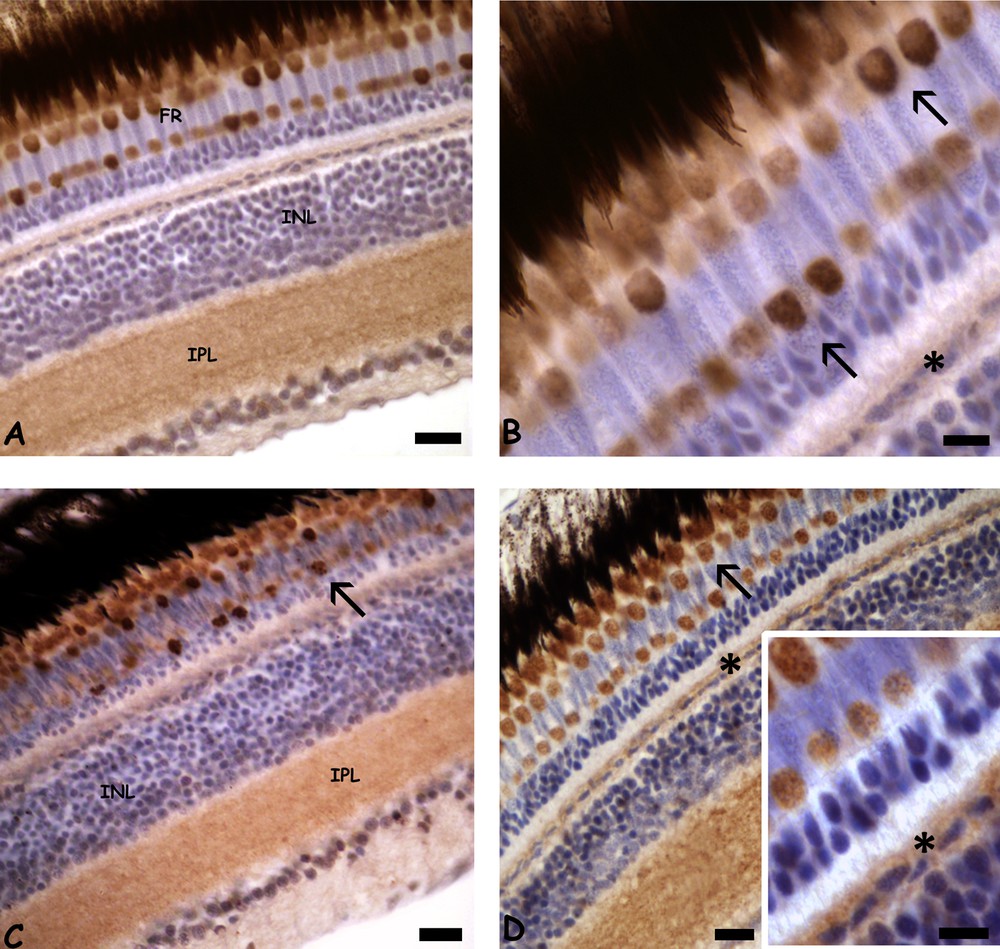
(Color online.) Immunolocalisation of metallothionein (MT) in retinae of Danio rerio control (A–B) or exposed to 0.3 (C) or 3.0 (D) mg/L cadmium chloride. A. MT in photoreceptors (FR) and in the inner plexiform layer (IPL). Unstained inner nuclear layer (INL). B. Detail of MT in cones inner segments (arrows). Note the faint stain on the horizontal cell cytoplasm (*). C. MT in cone inner segments (arrow) and IPL. D. Labelled cone inner segments (arrow) and horizontal cell cytoplasm (*). Inset: detail of labeled horizontal cells (*). Bars: A, C, D: 10 μm; B, inset: 5 μm. Counterstain with haemalum.
3.4 Effects of cadmium on the visual behavior response
Behavioral tests demonstrated that re-illumination with white or colored light does not induce an escape response in control animals (Fig. 6B). The animals swim regularly, entering in the bright sector an average of 27 times/min observation. This observation is not significantly different from that seen in untreated animals swimming under a diffuse white or colored light (Fig. 6A).

(Color online.) Escape response to a re-illumination with a white or a colored light in Danio rerio control (A, B) or exposed to 0.3 (C) or 3.0 (D) mg/L cadmium chloride. Blank (A) and control (B) animals pass in the bright sector of the tank an average of 27 times/min observation. No significant differences are observed between white and colored lights. In treated animals, a significant escape response is seen as increased avoidance of bright light at both cadmium concentrations and all wave-lengths. The response is more significant in animals treated at the lower, environmentally compatible dose of metal P < 0.05 (*).
In contrast, a significant escape response is observed after cadmium treatment. For the animals treated at the highest 3.0 mg/L dose, the number of passages into the bright sector decreases to an average of 12 times/min observation (Fig. 6D). After exposure to the lower environmentally realistic concentration, the effect is more pronounced as the animals enter the bright sector only about 5 times/min observation (Fig. 6C). No significant differences were observed in responses to different wavelengths of light.
4 Discussion
Retinal cells of Danio rerio exposed to cadmium contamination undergo significant dose-dependent deleterious changes. Induced cell death is seen in all cell types with a marked vacuolation in ganglion cell cytoplasm. Cytotoxic effects of cadmium have been demonstrated in different tissues (for example, in pituitary gland, [35]; liver, [36]; ovarian follicle cells, [37]), including zebrafish [38] and rat [39] nervous cells. The observed presence of cilia which are a hallmark of local mechanical, chemical or ionic trauma [40,41] adds to the evidence of physiological and biochemical cell stress.
Retinal cell death triggers an extensive repair and regeneration process that lasts for a few days up to several weeks. Müller cells, a subset of retinal macroglial cells, are able to dedifferentiate and function as neural stem cells [42,43]. These cells, located in the inner nuclear layer [44], are activated by pathogenic stimuli and injuries [45] to divide and migrate to replenish all other retinal layers [46,47]. We indeed have observed, especially at the higher dosage of contamination, the presence of both apoptotic cells and Müller cells with a particularly irregular shape.
Photoreceptor regeneration, however, is often accompanied by an improper localization of the newly differentiated cell. This is particularly evident for cones because of their complex tridimensional mosaic arrangement [48]. Whether this is a temporary condition that will eventually correct itself to a proper orientation is currently not known. It is also unclear whether these photoreceptors would function correctly.
The problems in repositioning, together with the consistent cell degeneration that we have seen indicate that the animals exposed to cadmium contamination suffer from a significant impairment of retinal functionality. The behavioral tests used in this study confirm this hypothesis as the exposed animals develop a hypersensitivity to light during the dark/light adaptation. This is the phase in which visual abilities are transferred from rods to cones [49] and therefore the observed escape from the light source supports the existence of functional alterations in cones. Differences observed in the response to different wavelengths are minimal, which suggests that single short, single long and double cones have probably undergone functional alteration. Interestingly, fish treated at the lower, “environmental” 0.3 mg/L cadmium dose actually have a greater escape response than those treated with a 10× higher dose. An explanation of this apparently anomalous behavior may be that the higher dose has activated more intense defense responses and/or recovery process. Our immunocytochemical analyses indicate that MTs does not increase in the two types of treatment significantly.
Based on our data, we can think that the recovery of visual acuity is due more to a regenerative process by the Müller cells rather than a defense mechanism through the MTs.
In conclusion, these experiments demonstrate that a realistic environmental concentration of cadmium can interfere with retinal structure and functionality resulting in modified visual performance of adult zebrafish. Most importantly, the greatest behavioral effect was observed at an environmentally relevant dose. This suggests that long-term exposure to levels of cadmium pollution, currently found in several rivers [32,50–53], is probably sufficient to impair a biological function so important to species in which vision is the foremost means of interaction with the environment. This effect would have obvious and severe implications for the survival of both individuals and, ultimately, the entire population exposed to contamination.
Author contributions
Conceived and designed the experiments: BA, CMM. Performed the experiments: BA, R. Crispino, R. Cerciello, PS, RP, CMM. Analyzed the data: BA, R. Crispino, R. Cerciello, PS, RP, CMM. Contributed reagents/materials/analysis tools: BA, CMM. Wrote the paper: BA, R. Cerciello, PS, CMM.
Disclosure of interest
The authors declare that they have no conflicts of interest concerning this article.
Acknowledgements
We would like to thank Dr. S. Sorbo and B. Saccomanno, C.I.S.M.E., Naples, for technical support.