1 Introduction
Heat shock proteins (HSPs) are a multigene family of molecular chaperones produced by cells in response to stressful conditions [1–5], mainly following thermal stress [6,7]. HSP70 is the most important and well-studied protein of all HSP family members, with an essential role in protein folding, disaggregation and degradation [8–11]. The massive literature about HSPs focuses mainly on photosynthetic eukaryotes [12–16], aquatic organisms [17,18] and model organisms [19–22]; in mammals, most recent studies aim to clarify the HSPs involvement in human diseases, such as neurodegenerative processes and cancer [23–28]. Terrestrial vertebrates such as reptiles and birds received less attention, both in terms of evolutionary and physiological studies. As regarding birds, studies mostly concern the effects of heat stress on broiler chickens [29–32].
In reptiles, HSP70 expression and functions have been correlated to thermal tolerance and adaptation [33–35]. Indeed, being ectotherm animals, reptiles are particularly susceptible to changes in environmental temperatures. Reptiles are globally distributed but show a relatively narrow range of viable temperatures, depending to the ecological niches of the species. Embryos of oviparous terrestrial vertebrates are considered more vulnerable to changes in environmental temperatures, because they have very limited capability to thermoregulate and are unable to move to more favourable environments.
It has been demonstrated that high temperatures as well as cold-stress, experimented by reptiles’ embryos during the development affect morphology and survival of hatchlings [36–39]. Many, if not all, studies on HSPs in reptiles aimed to clarify the role of these proteins in protecting embryos to thermal stress throughout the incubation period [35,36,40–44].
The wall lizard Podarcis siculus (Rafinesque-Schmaltz, 1810) is the most abundant reptile inhabiting countries of the Mediterranean basin. It is a seasonal breeder, and eggs deposition occurs in late spring-early summer, generally from May to July. Besides the environmental temperatures recorded in P. siculus reproductive season are quite warm (about 26–28/18–20 °C day/night temperatures), it has been demonstrated that the increase of incubation temperature at 30 °C caused the death of embryos; no survival was also observed if embryos were incubated early at 15 °C [36–38]. Nevertheless, P. siculus embryos of 15 days post-oviposition were able to survive following a 15 °C exposure for 5 days. However, this cold-shock induced many morphological alterations, as well as changes in gene expression, in developing embryos [36,37]: the analysis of HSP70 transcripts demonstrated that in early embryos (3 days post-oviposition) HSP70 mRNAs were present only in somites and spinal cords; 20 days post-oviposition, messengers were present in encephalon. Interestingly, the 15 °C cold-stressed embryos did not show significative changes in spatio-temporal expression of HSP70 gene expression [37].
It is well known that eggs of oviparous vertebrates accumulate maternal proteins to cope with the needs of the developing organisms; evidence exists on the role and contribution of HSPs transcripts and proteins during oogenesis and embryogenesis of some model organisms [45]. In this work, we decided to investigate the presence and localization of HSP70 protein during P. siculus embryogenesis, under natural thermal regime and following a non-lethal cold-shock.
2 Materials and methods
2.1 Animals
Gravid Podarcis siculus females (n = 10) were captured by noose or hand in rural sites outside of Napoli (Campania, Italy) during the reproductive period (from May to June). Animals were housed in a terrarium (115 cm × 35 cm × 48 cm) covered with sand on the bottom and contained hiding places, such as hollow bricks. The terrarium was maintained under conditions of natural temperature (26 °C day/18 °C night) and exposed to the natural photoperiodic conditions. Live mealworms were used to feed lizards (three times a week) and water was accessed freely. Daily check of the terrarium provided freshly laid eggs that were transferred to a terrarium in the same conditions of that used for adult specimens. Soil humidity was maintained by daily nebulisations of terrarium with water. Eggs were recovered at regular time intervals from deposition (5, 10 and 20 days post-oviposition, n = 8) and washed to remove soil traces. For the cold-shock treatment, 8 eggs at 20 days post-oviposition were exposed at a continuous temperature of 5 °C for 3 days. Embryos recovered from shells were checked for vitality (heart beating) and processed for cytological and molecular investigations.
All the procedures were performed following the Guidelines for Animal Experimentation of the Italian Department of Health and were organized in order to reduce stress and the number of animals used.
2.2 Immunohistochemistry
Whole lizard embryos were fixed in Bouin's solution for 6 h, dehydrated, incubated in melted paraffin at 58 °C, embedded and cut in 5–7 μm section using a microtome, according to routine protocols [46].
Immunohistochemical reactions were performed on deparaffinized embryo's sections using Novolink Polymer Detection Systems (RE7280-CE, Leica Biosystems). After antigen retrieval and quenching of endogenous peroxidase, sections were incubated overnight at 4 °C with HSP70 antibody (rabbit polyclonal antibody HSP70 (Santa Cruz Biotechnology, PA5-28202), diluted 1:200 in PBS. The sections were then incubated in Novolink polymer 1 h at RT and immunostaining was performed using 3,3′-diaminobenzidine (DAB) as chromogen. The sections were counterstained with hematoxylin, dehydrated, cleared in xylene, and mounted with a coverslip. To test the specificity of the reagents, some sections were incubated either with non-immune serum (1:10 in PBS) or 1% bovine serum albumin (BSA; Sigma). When the anti-HSP70 antibody was omitted, the staining was abolished. Images of sections were acquired using a Zeiss Axioskop microscope fitted with a TV camera.
2.3 Western blotting
Western blotting analyses were performed on total embryo homogenate to verify the amount of HSP70 in the different embryo's stages and after cold-stress. Embryos homogenates were obtained using RIPA buffer solution (150 mM NaCl, 50 mM Tris, 1% NP-40, 0.25% sodium deoxycholate, 0.1% SDS, pH 7.4) containing a cocktail of protease inhibitors (Sigma Aldrich Chemicals) and a polytron (KINEMATICA Polytron Model PT10-35 GT/PT 3100D Homogenizer, Fisher Scientific). Homogenates were centrifuged at 12,000 g for 10 min; the resulting pellet was discarded, and the supernatant used for analysis. Total protein content in supernatants was determined by the method of Hartree [47] using BSA as the protein standard.
Thirty micrograms of denatured proteins were electrophoresed in a 10% SDS – polyacrylamide gel as described by Laemmli [48], together with a pre-stained protein marker (ColorBurst Electrophoresis Marker m.w. 8–220 KDa, Sigma Aldrich). After the run, the proteins were transferred onto nitrocellulose membranes (Immobilon-P, Millipore, Switzerland) at 350 mA for 60 min. The membranes were blocked in blocking buffer solution (1 × TBS/1% Tween-20, 5% milk) for 60 min at room temperature and incubated overnight at 4 ̊C in milk/TBS-Tween buffer (1 × TBS/1% Tween-20, 2% milk) with the rabbit polyclonal antibody HSP70 (1:300) and with the mouse monoclonal antibody Actin (Santa Cruz Biotechnology sc-70319, 1:200), used as endogenous control. Membranes were washed 4 × 15 min. with TBS-Tween solution and incubated for 1 h at room temperature with a secondary antibody labelled with horseradish peroxidase, diluted in milk/TBS-Tween buffer (1 × TBS/1% Tween-20, 5% milk). The secondary antibodies used were: Anti-mouse: goat-anti-mouse, IgG-HRP, SC-2005 (Santa Cruz Biotechnology) 1:5000; Anti-rabbit: donkey-anti-rabbit, IgG-HRP, SC-2313 (Santa Cruz Biotechnology) 1:5000. Membranes were washed 4 × 15 min with TBS-Tween solution and revealed with a chemiluminescent method, using Luminol solution (final concentration 2.5 mM) in presence of cumaric acids (final concentration 0.4 mM) and H2O2 (final concentration 100 mM). The bands obtained were quantified using C-DiGit Chemiluminescent Western Blot Scanner (LI-COR).
3 Results
3.1 HSP70 localization in P. siculus developing embryos
Immunohistochemical analyses performed on 5 days post-oviposition embryos showed the protein present in the undifferentiated retina (Fig. 1A), in the outer proliferative layer, and in the cells of the undifferentiated encephalic vesicles (Fig. 1B). A clear positivity was also observed on the spinal cord, at the level of the ependymal cells and on the cytoplasm of the neurons forming the future grey matter (Fig. 1C). In the trunk the labelling was observed only on the developing kidney tubules (Fig. 1D).

Immunolocalization of HSP70 in 5 days Podarcis siculus embryos. A. Undifferentiated retina; diffuse staining (*) becoming particularly intense in the outer proliferating layer (arrow). Mitosis (small arrows). B. Undifferentiated encephalic wall; diffuse and moderate staining (*). C. Spinal cord with ependymal canal (e). Intense staining on ependymal cells (arrow) and neurons (small arrow) located in the future grey matter (g). Unstained white matter (w). D. Developing kidney tubules (t) with intensely labelled cells (*). Bars: 50 μm.
After 10 days from oviposition, the HSP70 protein was abundantly distributed in the nervous tissues (Fig. 2). In the eye, the protein was detected in the lens epithelium and in the retina, uniformly distributed in the cells of the undifferentiated distal region (Fig. 2B) and concentrated in ganglion cells and in the future amacrine cells. Possible labelling of the outer nuclear cells may have been masked by pigment cells (Fig. 2C). At this developmental stage, the encephalic vesicles started to organize and differentiate, and their walls variously thickened accordingly. An intense positivity was always observed on the ventricular zones (Fig. 2D–H); in addition, clearly positive neurons were observed in the developing septal and striatal structures of the telencephalon (Fig. 2D and E), in the mesencephalic tectum (Fig. 2F) and myelencephalic grey matter (Fig. 2H).

Immunolocalization of HSP70 in the head of 10 days Podarcis siculus embryos. A. Eye; labelling is present on lens epithelium (small arrows) and in the retina, both distal (dr) and proximal (pr). Vitreal humor (vh). B. Detail of distal retina with diffuse labelling (*). C. Detail of proximal retina with layering; labelling is on ganglion cells nuclei (gl) and on amacrine cells (small arrows). Inner plexiform layer (ipl) and bipolar cells (*) are unstained. D. Cross section of telencephalic vesicles; ventricles (v), septal intumescence (s), incipient ventricular ridge (*) and striatum (st). Labelling is concentrated on the ventricular zone (vz and arrows). E. Detail of the ventricular zone; note the intense staining (*) and the presence of several mitotic cells (small arrows). In the wall, several nuclei appear also labelled (*). F. Frontal section of diencephalon (d) and mesencephalon with tectum (t). Labelling is on ventricular zones (arrows) and tectum (t). G. Detail showing thalamic (vz) and hypothalamic (h) labelling. H. Myelencephalon with ventricle (v) and raphe (r). Labelling is in the ventricular zone (vz) and diffuse in the grey matter (*). Counterstain with haemalum: figure A and B; bars: 50 μm.
In the trunk, the protein localized in the pulmonary septa (Fig. 3A), in the spinal cord, in the grey matter, in the ventral ependymal cells and in the spinal ganglia (Fig. 3B). The protein was also present in the hepatic cords (Fig. 3C), in the undifferentiated mucosal cells of the gut (Fig. 3D) and in the renal tubules (Fig. 3E).
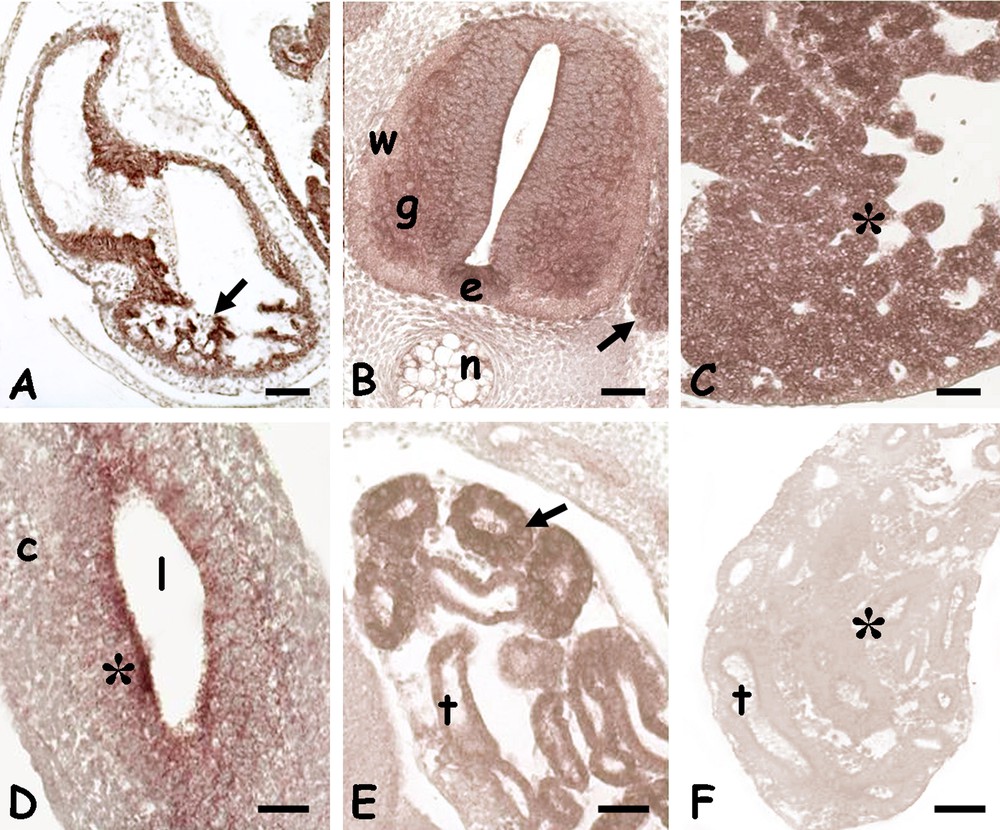
Immunolocalization of HSP70 in the trunk of 10 days Podarcis siculus embryos. A. Lung; labelled septa (arrow). B. Spinal cord; labelled grey matter (g), ventral ependymal cells (e) and spinal ganglia (arrow). Unlabelled white matter (w), notochord (n). C. Liver; labelled hepatic cords (*). D. Gut; moderate labelling on mucosal cells (*). Unlabelled connective (c), lumen (l). E. Developing kidney tubules (t) with intensely labelled cells (*). F. Negative control; unstained kidney tubules (t) (*). Bars: 50 μm.
In embryos of 20 days post-oviposition, most structures were well defined. In the head, the eyes (Fig. 4A) had a large vitreal humour and the retina was completely layered. The HSP70 was observed in the nuclei of ganglion cells and, to a lesser extent, in the amacrine cells (Fig. 4A and B). In all the encephalic vesicles an intense staining was observed on the periventricular zone. In addition, the protein was also localised in the telencephalic hemispheres, in dispersed nuclei of the incipient cortex and in the dorsal ridge (Fig. 4C and D), in the midbrain, in the cells destined to form the optical roof (data not shown) and in the oblongata, in ventro-lateral commissural fibres (Fig. 4E).
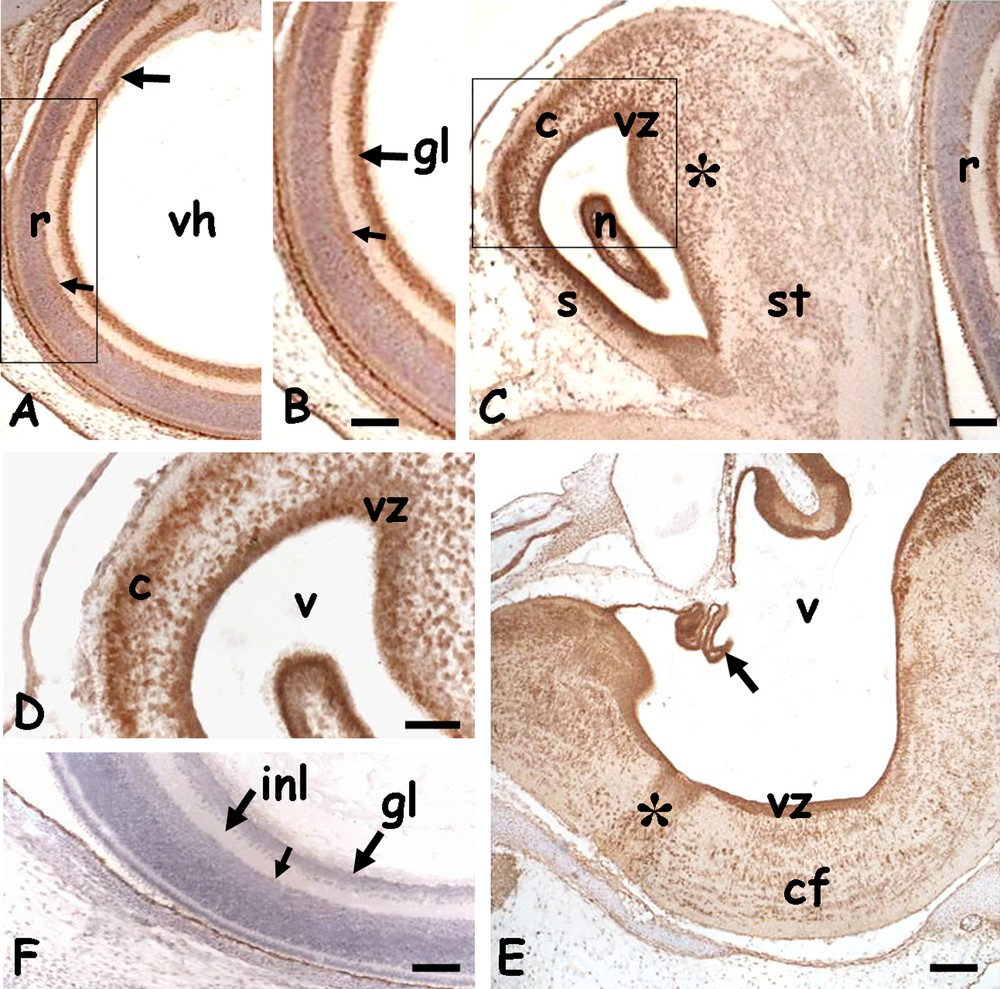
Immunolocalization of HSP70 in the head of 20 days Podarcis siculus embryos. A. Eye; layered retina (r) with labelled nuclei of the ganglion (arrow) and amacrine (small arrow) cells. Vitreal humor (vh). B. Detail. C. Telencephalon; intense staining on the periventricular zone (vz) and in dispersed cortical (c) and dorsal ridge's (*) nuclei. Septum (s), striatum (st), nucleus sphericus (n). D. Detail. E. Myelencephalon with intensely labelled ventricular zone (vz) and plexus chorioideus (arrow). Labelling is also on dispersed nuclei present in the raphe (*) and commissural area (cf). V: IV ventricle. F. Negative control; retina with gangliar (gl) and inner plexiform (ipl) layers. Amacrine cells (small arrow). Counterstain with haemalum: figure A–C, F; bars: 50 μm.
In the spinal cord, the protein was detected in the grey matter, in the nerve roots (Fig. 5A) and spinal ganglia (Fig. 5F). A significant labelling was also observed in the mucosa of the developing gut villi (Fig. 5B and D), in heart ventricular fibres (Fig. 5C), in the hepatic parenchyma (Fig. 5D) and kidney tubules. Negative controls were always unlabelled (Figs. 3D, 4F, 5G).
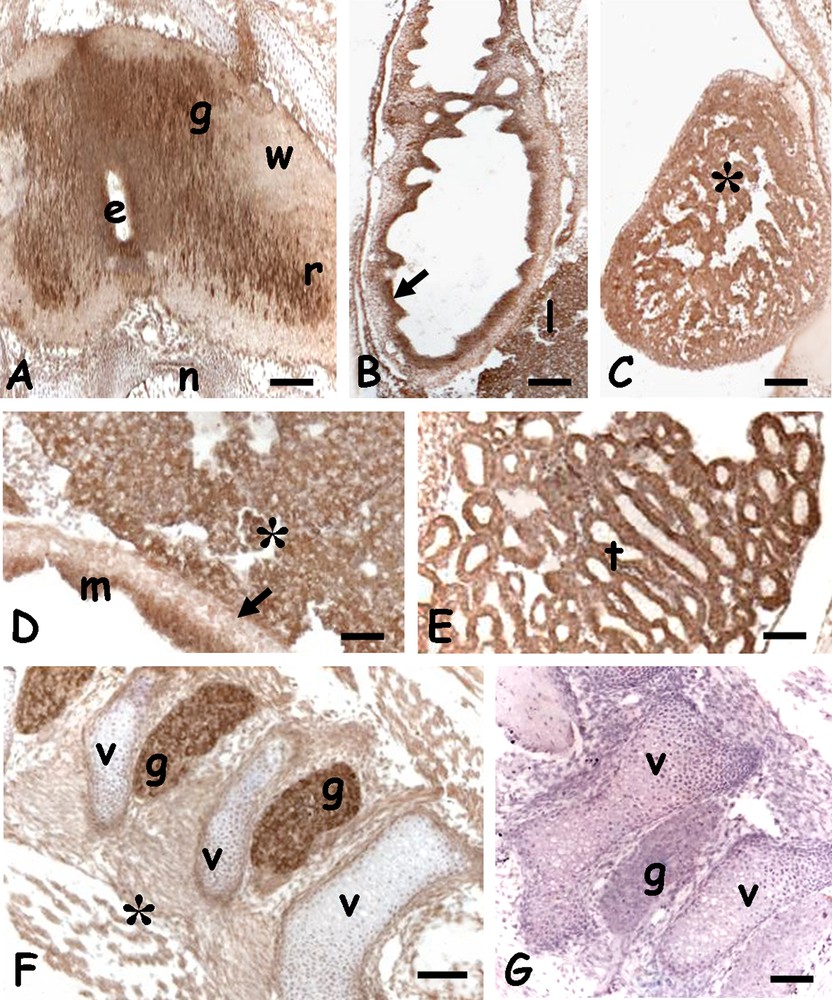
Immunolocalization of HSP70 in the trunk of 20 days Podarcis siculus embryos. A. Spinal cord; labelled grey matter (g) and ventral nerve root (r). Ependymal canal (e), unlabelled white matter (w), notochord (n). B. Gut with labelled mucosa (arrow). Liver (l). C. Heart with labelled musculature (*). D. Detail of labelled mucosa (m) and liver cords (*). Unlabelled muscolaris mucosae (arrow). E. Labelled kidney tubules (t). F. Parasagittal section of vertebral column; labelled spinal ganglia (g). Unlabelled vertebrae (v) and connective tissues (*). G. Negative control; unstained vertebrae (v) and spinal ganglia (g). Bars: 50 μm.
3.2 HSP70 localization in P. siculus embryos under cold-stress condition
Eight embryos at 20 days post-oviposition were incubated for 3 days at a continuous thermal regime of 5 °C. At the end of the treatment, macroscopic observations showed the viability and microscopic analysis the absence of substantial changes in embryos morphology. Immunohistochemical analysis demonstrated that the distribution of the HSP70 protein did not change following the exposure of the developing embryo to a strong thermal shock (from 26 to 5 °C) (Fig. 6).
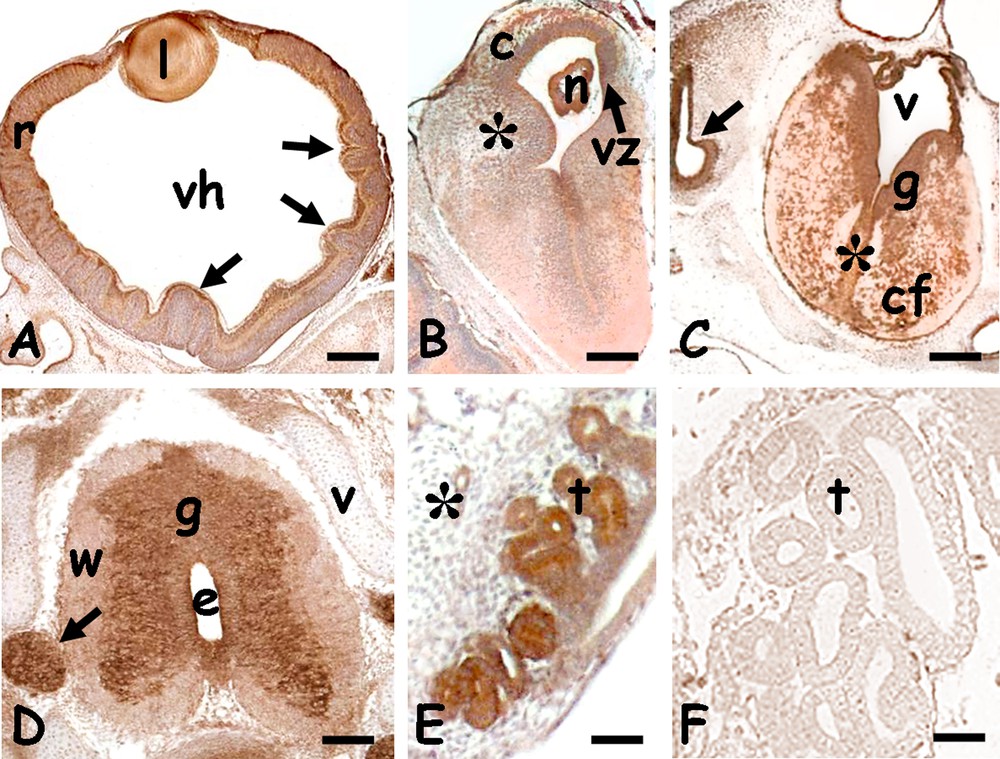
Immunolocalization of HSP70 in 20 days cold-stressed Podarcis siculus embryos. A. Eye; labelled lens (l) and retina (r) with folds (arrows). Vitreal humor (vh). B. Telencephalon; intense staining on the periventricular zone (vz) and in dispersed cortical (c) and dorsal ridge's (*) nuclei. Nucleus sphericus (n). C. Myelencephalon with intensely labelled grey matter (g). Labelling is also on dispersed nuclei present in the raphe (*) and commissural area (cf). V: IV ventricle, optic vesicle (arrow). D. Spinal cord; labelled grey matter (g) and spinal ganglia (arrow). Unlabelled white matter (W) and vertebrae (v). Ependymal canal (e). E. Labelled kidney tubules (t). F. Negative control; unstained kidney tubules. Bars: 50 μm.
Indeed, in the eye, the protein was found in the lenticular epithelium, in ganglion cells and in the inner and outer nuclear layers of the retina (Fig. 6A); interestingly, the labelling was evident even in the areas of the retina folded as a consequence of the cold treatment. In the encephalic vesicles the labelling was more evident on the periventricular zone, and weakly diffused on nuclei of the telencephalic cortex and dorsal ridge (Fig. 6B) and those dispersed in the myelencephalic white matter (Fig. 6C).
Spinal cord of cold-shocked embryos showed the same morphology and immunoreactivity of control embryos, with the labelled butterfly-shaped grey matter and spinal ganglia; the white matter was negative (Fig. 6D). In the trunk no difference in labelling localization was observed with respect to untreated embryos (renal tubules, Fig. 6E; other organs not shown).
3.3 HSP70 content in P. siculus embryos
Western blotting analysis was performed on homogenates obtained from embryos incubated for 10 and 20 days post-oviposition according to a natural thermal regime (26 °C day, 18 °C night) and from embryos maintained for 20 days at the natural thermal regime and then subjected to a cold-shock stress (3 days at 5 °C). Results from three independent homogenates for each sample demonstrated that the amount of HSP70 in whole embryo did not change during development and following cold-shock (Fig. 7).

Western blotting analysis of HSP70 expression in control and cold-stressed P. siculus embryos. Membranes were stained with the anti-HSP70 and the anti-actin IgG. Bars represent the main relative levels of HSP70 protein in P. siculus embryos, expressed in arbitrary units (a.u.), obtained by using the C-DiGit Chemiluminescent Western Blot Scanner (LI-COR). The endogenous actin was used for normalization. Lane 1, total homogenate from embryos of 10 days post-oviposition; lane 2, total homogenate from embryos of 20 days post-oviposition; lane 3, total homogenate from embryos of 20 days post-oviposition incubated for additionally 3 days at 5 °C (cold-stressed embryos).
4 Discussion
The present study provides a further understanding of HSP70 involvement in Podarcis siculus development. The results herein described demonstrate that this protein is constitutively present in the early developmental stages. In particular, after 5 days from oviposition the HSP70 is distributed in all the Central Nervous System (CNS) and in trunk organs; the protein is recorded in the same organs also after 10 and 20 days from oviposition. In the CNS, the protein is mostly abundant in periventricular cells and in the grey matter areas. This protein localization only partially overlaps with that determined for transcripts [36–38]. Indeed, according to previous in situ hybridization analysis performed during P. siculus embryogenesis, HSP70 mRNAs are detectable in CNS grey matter and in the developing eyes, but are absent in trunk organs, as kidney and liver [36–38]. Such data allows us to hypothesize that the HSP70 highlighted in the embryonic tissues is at least in part attributable to the maternal proteins stored in the egg before fertilization. In addition, data collected during this study show that the HSP70 protein content does not increase following thermal shock; this result is in agreement with that observed in P. siculus embryos for the HSP70 gene expression, that is not cold-induced [37,38].
A specific member of HSP superfamily, called HSP30, was found in fish, amphibians, reptiles and birds, but is lacking in mammals [49]. The HSP30 gene is transcribed in response to thermal shock and other stress factors, such as heavy metals, all able to enhance in the cells the amount of damaged proteins [49–51]. The HSP30 shows a tissue-specific expression during embryogenesis [49,52–54]; in frog embryos, heat shock induces a HSP30 stage-specific expression [49,55].
In light of the above, it is possible to hypothesize that in P. siculus embryos the HSP70 represents a constitutive molecular chaperone, playing an important role for the correct development of the animal and that should be better called Heat Shock Cognate 70 (HSC70); other members of the HSP family, such as the non-mammalian HSP30, could be activated following physical and/or chemical stresses.
The HSP30 gene expression and synthesis were intensively studies in Xenopus [49]; in reptiles, the HSP30 gene was identified in some species [49], but the putative HSP30 protein was immunodetected only in the lizard Japalura mitsukurii kidney cell culture exposed to heat shock [56]. It has been demonstrated that P. siculus embryos are particularly susceptible to changes in incubation temperature, and that temperature oscillation can elicit serious morphological alterations, gene inhibition and, in some cases, can lead to the embryo death [36–38]. In these embryos, similar effects are also caused by other stressors, such as cadmium [57–59], despite the fact that the embryonic tissues show the presence of the transcripts encoding the metallothionein, an important cytoprotective protein involved in cadmium detoxification [58,60]. Further research is needed to establish the presence and activation of other HSPs, such as HSP30, during the different stages of lizard embryonic development, under normal conditions and following stressful conditions. In addition, further studies could shed new light on why these proteins that should be cytoprotective fail to protect embryos from chemical and physical insults.
Acknowledgments
This work was supported by the University of Napoli Federico II, Italy. The study was performed in strict accordance with the criteria established by the National Institutes of Health. The Committee on the Ethics of Animal Experiments of the University of Naples Federico II approved the protocol (ID: 2013/0096988). The animals were kept according to the authorization by the Ministry of the Environment and Protection of Land and Sea (also known as MATTM; permit 0004363/PMN-2015).