1. Introduction
Radiotherapy (RT) is one of the most important methods for cancer treatment. An estimated 2.9 million patients will be diagnosed with cancer in the EU-28 in 2025, of whom approximately 50% will receive external beam radiotherapy [1]. The technological advances in RT in recent decades have led to improved tumor dose conformation, thus reducing the dose to organs-at-risk [2]. However, adverse effects of RT are still common, and remain the main limitation of RT. Consequently, the tolerance of normal tissue to RT compromises effective treatment for radioresistant tumors.
RT is going through a paradigm shift driven by the ever-increasing evidence of the importance of the so-called “non-targeted” effects of radiation on the biological response, including cell signaling [3], and vascular [4], stromal, and immunological changes [5]. Interestingly, these effects can be activated and/or modulated by changing the physical parameters of the radiation, i.e., the dose delivery methods [6]. Proton minibeam radiation therapy (pMBRT) [7] is a novel cancer therapy that promises to overcome the limitation of RT posed by side effects. pMBRT is based on a distinct dose delivery method using a strong spatial modulation of the dose to create alternating regions of high and low doses, in contrast to the homogeneous dose distributions used in standard RT (Figure 1). Minibeam radiation therapy (MBRT) originated [8] at large synchrotron sources [9], facilities that provide intense kilovoltage X-ray beams with negligible beam divergence. The irradiation is performed with arrays of parallel and thin beams (0.5–1.0 mm wide). As normal tissue radiosensitivity depends on the volume irradiated [10], the use of such narrow beams may allow the use of higher and potentially curative doses in difficult-to-treat cancers. This contrasts with some other spatially fractionated RT techniques, such as GRID [11] and Lattice therapy [12], which are mainly limited to palliative treatment of bulky tumors.
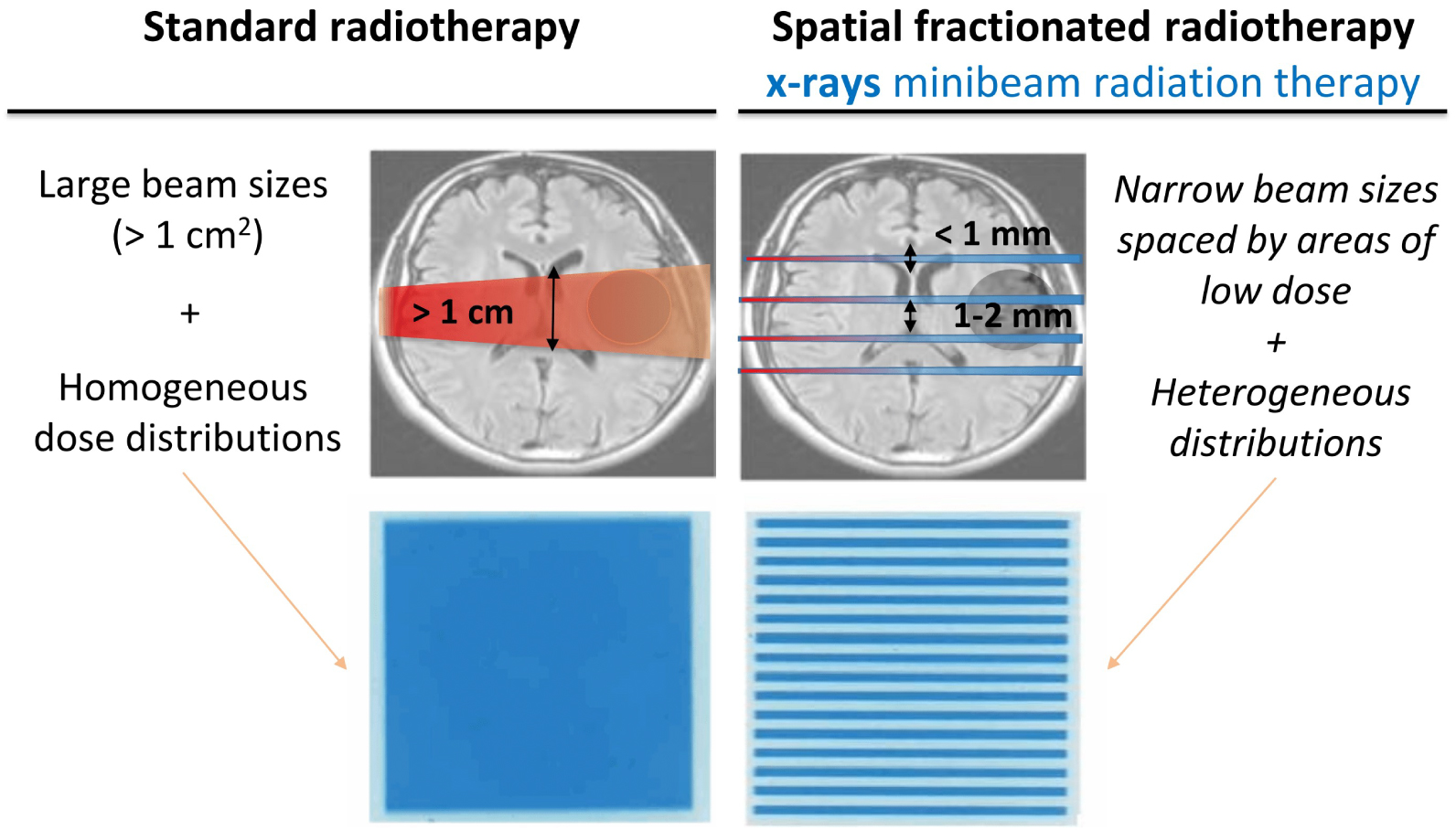
Scheme illustrating the differences between standard RT and SFRT.
Indeed, numerous experiments have shown an increase in the therapeutic index in radioresistant tumors. X-rays MBRT offers an extraordinary normal tissue sparing at very high doses (higher than 50 Gy in one fraction) [8, 13, 14] accompanied by a remarkable tumor control effectiveness [15, 16].
In recent years, X-rays MBRT has been implemented outside large synchrotrons using low-cost equipment, such as small animal irradiators [17, 18]. However, X-rays MBRT uses kV photon beams, which are inadequate for deep-seated tumor treatments, to reduce lateral scattering. The use of more energetic X-rays (megavoltage beams) would lead to reduced spatial modulation, thereby reducing the treatment benefits.
The use of protons in combination with MBRT allows to fully profit from the advantages of the spatial fractionation of the dose, which paves a direct path for optimal treatments in the future. Termed proton minibeam radiation therapy (pMBRT) [7], this method offers several advantages over X-ray-based MBRT, including negligible dose deposition in normal tissue after the Bragg peak, and the possibility of producing a uniform dose at depth, while maintaining modulation on the beam entrance side (Figure 2). This represents an advantage over X-rays MBRT, where multiple interlacing fields are usually needed to achieve target dose homogenization, which is not only more error-prone but also technically more difficult.
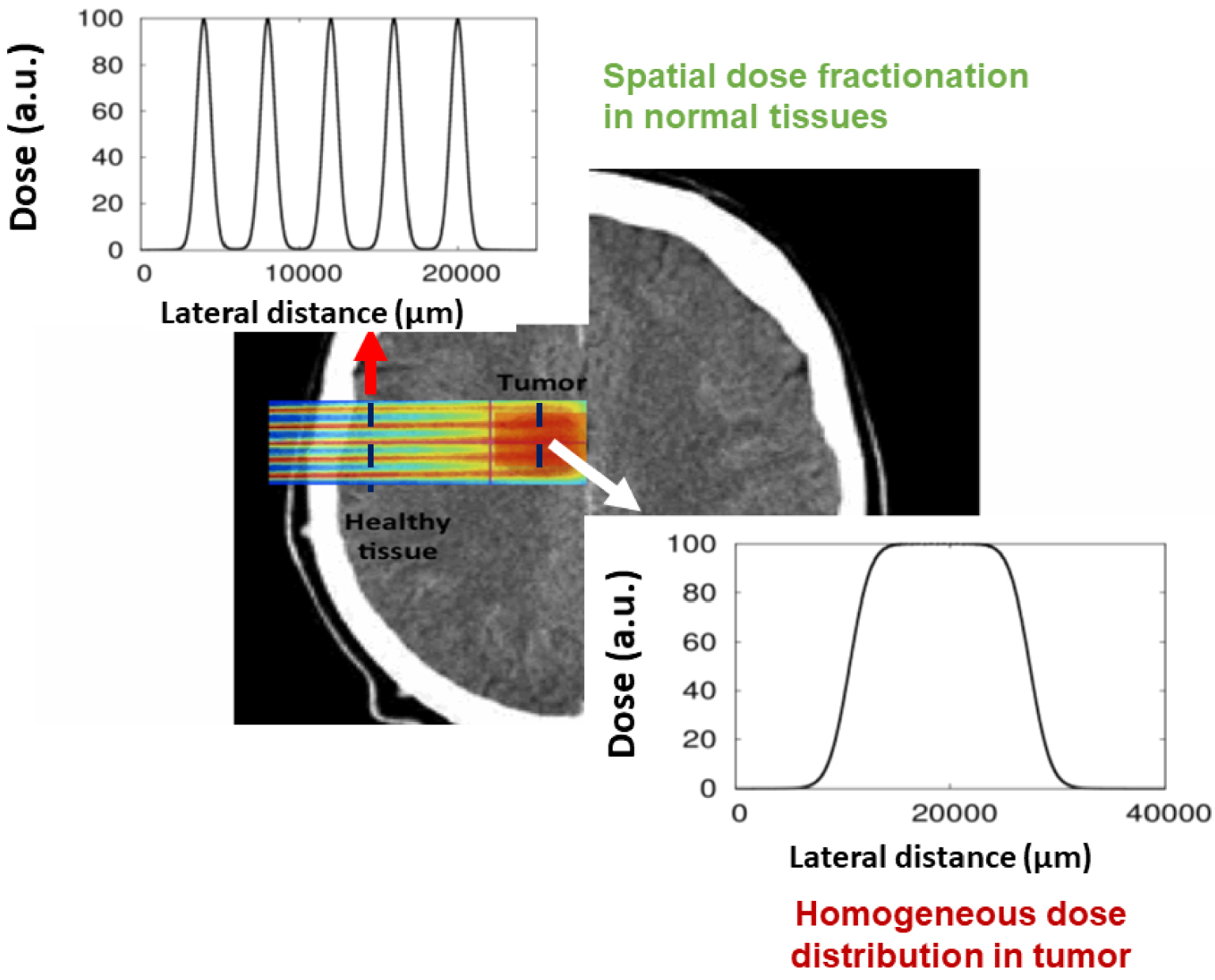
Dose distributions in pMBRT.
The biological evaluations of pMBRT performed thus far have attested a remarkable reduction in normal tissue toxicity [19, 20, 21, 22] and equivalent, or even superior, tumor control compared to standard proton therapy (PT) [23, 24]. In the next sections the state of the art and perspectives of pMBRT will be reviewed.
2. Proton minibeam radiation therapy: state of the art
The first worldwide technical implementation of pMBRT at a clinical center [25] was conducted in 2014 at the Institut Curie-Orsay proton therapy center (ICPO). This first implementation was performed at one of the passive beamlines of the ICPO using mechanical collimators. In 2015, Dilmanian et al. [26] confirmed the physical feasibility of the technique with a similar implementation. Technological advances in proton minibeam generation at clinical centers include its implementation at a pencil beam scanning system with optimized multislit collimators [27], a scanning dynamic collimator for spot-scanning proton minibeam production [28], and a novel nozzle design for magnetically-focused minibeams [29].
A series of radiobiological studies have demonstrated remarkable normal tissue toxicity reduction provided by pMBRT compared to standard PT.
Initially, reduced cell viability and an increased inflammatory response were observed after homogenous broad-beam irradiation, as compared to pMBRT irradiation, in a three-dimensional (3D) human skin model [20]. Mouse ears were irradiated either with proton minibeams or proton broad-beam irradiation. The same deposited dose (60 Gy) resulted in a significantly higher degree of edema, erythema, or desquamation in animals receiving broad-beam irradiation compared to those receiving pMBRT [19].
In another pioneering experiment, the remarkable reduction in neurotoxicity provided by pMBRT was demonstrated [22]. The whole brains (except the olfactory bulb) of two groups (n = 8/each) of normal rats (7 weeks old) were irradiated: (i) the first group received a seamless (standard) proton irradiation, with high doses (25 Gy in one session); (ii) the second group received pMBRT irradiation with peak doses of 58 Gy per fraction (corresponding to the same average dose of 25 Gy). After a 6-month follow-up, a longitudinal magnetic resonance imaging (MRI) study using a 7-T small-animal MRI scanner, together with histological and immunochemical analyses, was conducted. Rats treated with conventional proton irradiation presented grade 3 skin damage (moist desquamation) and extensive brain damage, including necrosis, demyelination, astrogliosis, and microglial activation. On the contrary, pMBRT treatment resulted in no significant damage [22] (Figure 3, left). It has also been demonstrated that pMBRT has no significant impact on cognitive, motor, or emotional processes after whole rat brain irradiations [21].
This reduction in neurotoxicity observed with pMBRT compared to standard RT may improve the therapeutic index in cases that have good rates of tumor control but are accompanied with substantial side effects, such as pediatric astrocytoma. It also opens the door for a more efficient treatment of radioresistant tumors, such as high-grade gliomas (GBM), which remain one of the most challenging diagnoses in clinical oncology.
Indeed, in a previous study in glioma-bearing rats, pMBRT showed a tumor-control efficacy equal or superior to that of standard RT, and without the side effects observed in different rat glioma models, thus opening up the possibility of more aggressive irradiation schemes [23, 24, 30]. Besides, in some configurations, a 70% long-term survival rate ( >6 months, free of tumor) was obtained in the pMBRT group [24]. See Figure 3, right. The mean survival time was significantly higher than that in the standard PT group, despite the heterogeneous target dose coverage. Moreover, tumor sterilization was even achieved in cases where the beam spacing was large (3.5 mm) and large areas of the tumor received low (non-tumoricidal) doses [30]. This contradicts the classical paradigm of RT requiring the deposition of a lethal dose in every tumor cell for tumor ablation. Moreover, the fact that large areas (or even the whole brain) could be irradiated without significant side effects would allow the infiltrative nature of the tumor to be overcome, one of the major difficulties to be faced in glioma treatment.
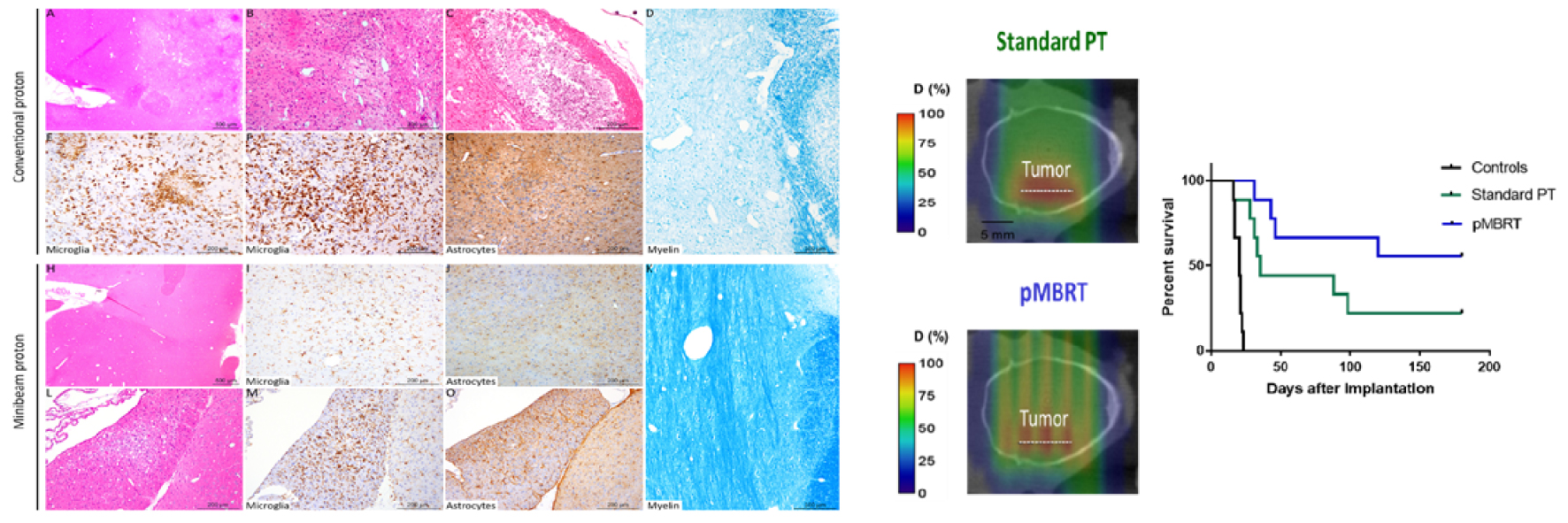
An illustration of the net gain in the therapeutic index of pMBRT. Left: Minimum toxicity in pMBRT. Histopathological and immunohistochemical analyses revealed substantial damage in the conventional group (A–G). Conventional irradiation: (A) Multifocal to coalescing lesions characterized by (B) edema, necrosis, and gliosis. (C) More severe lesions with cavitation and mineralization. (D) Destruction of myelin was also observed. (E, F) Microglial activation and nodules (microgliosis). (G) Astrocyte activation with a marked increase in the GFAP immunolabeling (astrogliosis). Minibeam irradiation: (H) No lesions were observed in most of the rats (7 out of 8), with (I, J) normal microglial and astrocytic networks, and (K) normal myelin organization. For just one rat: (L) One infammatory microscopic inflitrate and mild neuropil destruction was observed, associated with focal microscopic (M) microgliosis and (O) astrogliosis. Taken from [22]. Center: Dose distributions received by the tumor-bearing rats. Right: Tumor control. Survival curves of tumor-bearing rats: controls (black line); standard PT (green); pMBRT with quasi-homogeneous dose in the target (blue). An enhanced lifespan is observed. Taken from [24]. Masquer
An illustration of the net gain in the therapeutic index of pMBRT. Left: Minimum toxicity in pMBRT. Histopathological and immunohistochemical analyses revealed substantial damage in the conventional group (A–G). Conventional irradiation: (A) Multifocal to coalescing lesions characterized by (B) edema, ... Lire la suite
The studies conducted to date have used a single-fraction irradiation scheme. Standard RT usually employs temporal fractionation of the dose to profit from both the reoxygenation of the tumor and redistribution of cells to more sensitive phases of the cell cycle (late G2 and M phase) [31]. Local tissue toxicity is also reduced due to the increased time available for the repair of sublethal damage, in addition to the repopulation of cell fractions [31]. Thus, temporal fractionation could also help and sum up to the inherent benefit of the spatial fractionation of the dose for normal tissue preservation. A recent study reported, for the first time, the response of glioma-bearing rats to a temporal fractionation in pMBRT delivered under a crossfire geometry. A significant increase in the median survival time was obtained when the dose was delivered over more than two sessions as opposed to in a single fraction. Moreover, 83% of long-term survivors had a total lack of tumoral cells, which is 3.3 times higher than that observed with conventional broad-beam irradiation, without excess of toxicity. This result could facilitate the path toward pMBRT treatments.
Compared to direct damage by ionizing radiation, pMBRT seems to activate different biological mechanisms; with the immune system already observed to play an important role [32]. Other factors may include cell signaling effects, i.e., bystander-like effects [33], and the preferential effect on the tumoral versus normal vasculature [4]. The participation of these phenomena needs to be further investigated with a comprehensive approach. Additionally, it remains unclear how these effects are translated when the spatial dose distribution is modified. Indeed, no systematic evaluation of the influence of the beam width and spacing on the tissue response to spatially fractionated RT has been performed.
3. Perspectives: toward clinical trials
The ultimate goal is to translate and reproduce the findings of pMBRT from small animal experiments into the clinic.
Indeed, ten patients with large tumors have already been treated with proton GRID therapy (1-cm wide beams) [34] with palliative intent. These patients had tumors that could not be treated with photons, standard broad-beam irradiation, or photon GRID due to the tolerance of normal tissues. The improvement rate in terms of symptom relief and partial tumor regression was 80% for all patients, while acute side effects (grade 1/2) appeared in 50% of the patients, all of which were well-tolerated.
The experimental data gathered so far indicate that pMBRT can increase the therapeutic index, allowing the move from palliative to curative treatments. Patients thought to benefit the most from the advantages of pMBRT include those suffering from lymphomas, low-grade gliomas, or benign lesions, such as meningioma; these patients may benefit from the reduction in side effects while maintaining a comparable local control in diseased tissue. Moreover, in patients who are affected by radiation-resistant tumors, such as GBM, osteosarcoma, chordoma, or chondrosarcoma, pMBRT may facilitate higher potentially curative doses in the tumor. The first therapeutic trials could include primitive brain tumors with a poor prognosis, such as recurrent gliomas.
The first theoretical investigation was conducted on possible treatment plans for two brain tumors, glioma and meningioma, in virtual patients derived from anonymized human patient imaging records. The pMBRT treatment plans were compared to standard (seamless) proton therapy plans, and they met the criteria recommended for clinically acceptable dose-volume histograms in organs-at-risk. Satisfactory homogeneity was achieved in the target, while a similar level of spatial dose modulation was obtained in normal tissues as that observed in preclinical studies [35]. Future research will be focused on unraveling the underlying mechanisms, how these effects are translated when the spatial distribution is modified, and linked to that, determining the best way to prescribe the dose in pMBRT, i.e., in terms of peak, valley, average dose, or potentially, a novel metric.
4. Conclusion
pMBRT is a novel cancer therapy approach that promises a high therapeutic index in difficult-to-treat cases, such as GBM. pMBRT is considered to be particularly appealing for use in pediatric oncology due to its remarkably low neurotoxicity. pMBRT offers the best balance between the broad beams employed in GRID and lattice therapy, both of which are currently limited to palliative treatments, and the extreme conditions of microbeam radiation therapy which difficult the passage of this latter technique to patients’ treatments. The alliance of protons and MBRT allows the physical advantages of protons for therapy to be combined with the biological advantages of MBRT, promising an optimal treatment. Indeed, strong spatial dose modulation in pMBRT was shown to lead to a significant reduction in side effects while providing equivalent or superior tumor (high-grade glioma) control than standard homogeneous irradiation in small animal experiments. Despite its infancy, the biological data gathered thus far challenge the classical paradigm in RT and encourage the preparation of phase I clinical trials. This preparation is ongoing at Institut Curie.
Conflicts of interest
Authors have no conflict of interest to declare.
Acknowledgments
Y. Prezado warmly thanks the work of the current and former members of her team “New Approaches in Radiotherapy”, the personnel of the Orsay proton therapy centre and the Experimental Radiotherapy platform of Institut Curie. Y. Prezado acknowledges the funding from European Research Council (ERC) under the European Union’s Horizon 2020 research and innovation programme (grant agreement No 817908).
French version
1. Introduction
La radiothérapie (RT) est l’une des méthodes les plus importantes pour le traitement du cancer. On estime que 2,9 millions de patients recevront un diagnostic de cancer dans l’UE-28 en 2025, dont environ 50% recevront une radiothérapie externe [1]. Les progrès technologiques réalisés dans le domaine de la radiothérapie au cours des dernières décennies ont permis d’améliorer la conformation de la dose reçue par la tumeur, réduisant ainsi la dose reçue par les organes à risque [2]. Cependant, les effets indésirables de la RT sont encore fréquents et restent la principale limitation de la RT. Par conséquent, la tolérance des tissus normaux à la RT compromet l’efficacité du traitement des tumeurs radiorésistantes.
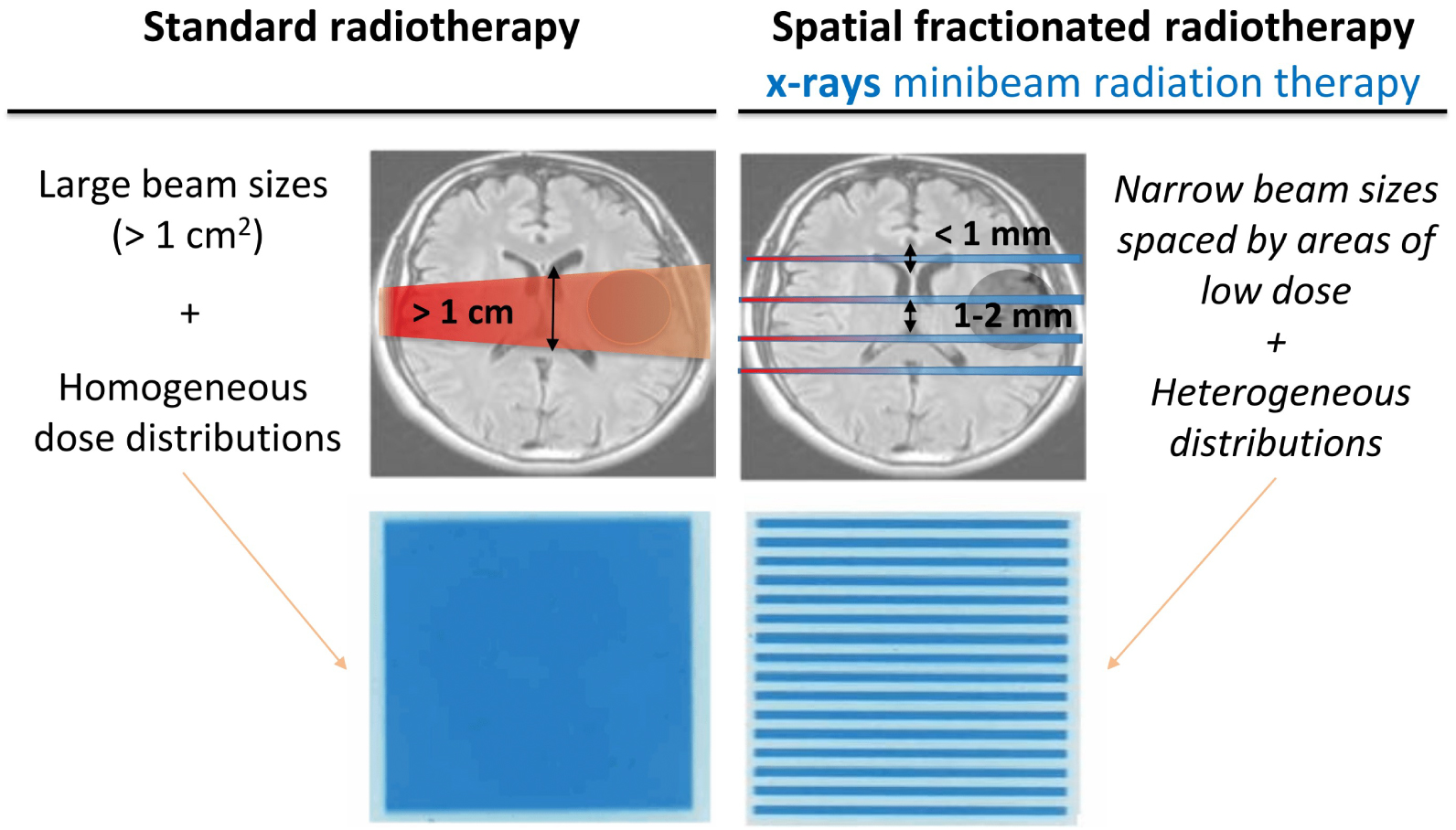
Schéma illustrant les différences entre la RT standard et la SFRT.
La radiothérapie est en train de changer de paradigme en raison des preuves toujours plus nombreuses de l’importance des effets dits « non ciblés » des rayonnements sur la réponse biologique, notamment la signalisation cellulaire [3] et les modifications vasculaires [4], stromales et immunologiques [5]. Il est intéressant de noter que ces effets peuvent être activés et/ou modulés en modifiant les paramètres physiques de l’irradiation, c’est-à-dire les méthodes de délivrance de la dose [6]. La radiothérapie par mini-faisceaux de protons (pMBRT) [7] est une nouvelle thérapie anticancéreuse qui promet de surmonter les limitations de la RT dues aux effets secondaires. La pMBRT est basée sur une méthode d’administration de dose distincte utilisant une forte modulation spatiale de la dose pour créer des régions alternées de doses élevées et faibles, contrairement aux distributions de dose homogènes utilisées dans la RT standard (Figure 1). La radiothérapie par mini-faisceaux (MBRT) est née [8] dans les grandes sources synchrotron [9], qui sont des installations qui fournissent des faisceaux de rayons X intenses de kilovoltage avec une divergence de faisceau négligeable. L’irradiation est réalisée à l’aide de réseaux de faisceaux parallèles et fins (0,5–1,0 mm de large). Comme la radiosensibilité des tissus normaux dépend du volume irradié [10], l’utilisation de faisceaux aussi étroits peut permettre d’utiliser des doses plus élevées et potentiellement curatives dans les cancers difficiles à traiter. Cela contraste avec d’autres techniques de RT fractionnée dans l’espace, telles que le GRID [11] et la thérapie Lattice [12], qui sont principalement limitées au traitement palliatif de tumeurs volumineuses.
En effet, de nombreuses expériences ont montré une augmentation de l’index thérapeutique dans les tumeurs radiorésistantes. La MBRT aux rayons X offre une extraordinaire épargne des tissus normaux à des doses très élevées (plus de 50 Gy en une fraction) [8, 13, 14] accompagnée d’une remarquable efficacité de contrôle des tumeurs [15, 16].
Ces dernières années, la radiothérapie par mini-faisceaux de rayons X a été mise en œuvre en dehors des grands synchrotrons à l’aide d’équipements peu coûteux, tels que des irradiateurs pour petits animaux [17, 18]. Cependant, le MBRT à rayons X utilise des faisceaux de photons de kV afin de réduire la diffusion latérale mais qui sont inadéquats pour le traitement de tumeurs profondes. L’utilisation de rayons X plus énergétiques (faisceaux de Mégavoltage) entraînerait une réduction de la modulation spatiale, ce qui réduirait les avantages du traitement.
L’utilisation de protons en combinaison avec la MBRT permet de profiter pleinement des avantages du fractionnement spatial de la dose, ce qui ouvre la voie à des traitements optimaux dans le futur. Appelée radiothérapie par mini-faisceaux de protons (pMBRT) [7], cette méthode offre plusieurs avantages par rapport à la MBRT à base de rayons X, notamment un dépôt de dose négligeable dans les tissus normaux après le pic de Bragg, et la possibilité de produire une dose uniforme en profondeur, tout en maintenant la modulation du côté de l’entrée du faisceau (Figure 2). Cela représente un avantage par rapport à la MBRT aux rayons X, où de multiples champs entrelacés sont généralement nécessaires pour obtenir une homogénéisation de la dose cible, ce qui est non seulement plus propice aux erreurs mais aussi plus difficile techniquement.
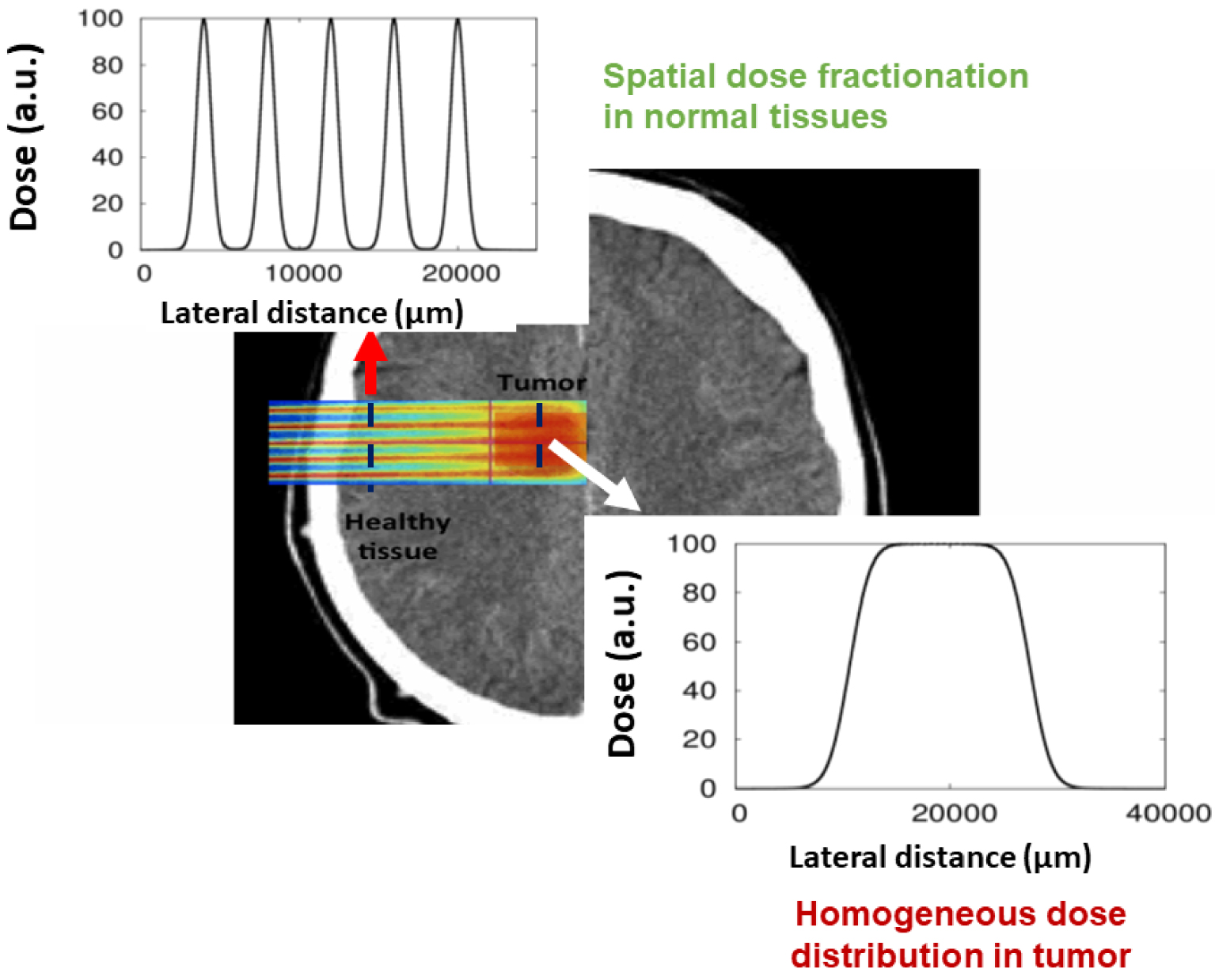
Distributions de dose en pMBRT.
Les évaluations biologiques de la pMBRT réalisées jusqu’à présent ont attesté d’une réduction remarquable de la toxicité des tissus normaux [19, 20, 21, 22], et d’un contrôle tumoral équivalent, voire supérieur, par rapport à la protonthérapie (PT) standard [23, 24]. Les sections suivantes présentent l’état de l’art et les perspectives de la pMBRT.
2. Radiothérapie par mini-faisceaux de protons : état de l’art
La première mise en œuvre technique mondiale de la pMBRT dans un centre clinique [25] a été réalisée en 2014 au centre de protonthérapie de l’Institut Curie-Orsay (ICPO). Cette première mise en œuvre a été réalisée sur l’une des lignes de faisceau passives de l’ICPO en utilisant des collimateurs mécaniques. En 2015, Dilmanian et al. [26] ont confirmé la faisabilité physique de la technique avec une implémentation similaire. Les avancées technologiques dans la production de mini-faisceaux de protons dans les centres cliniques incluent sa mise en œuvre dans un système de « pencil beam » avec des collimateurs multi-fentes optimisés [27], un collimateur dynamique [28], et une nouvelle conception de « nozzle » pour les mini-faisceaux à focalisation magnétique [36].
Une série d’études radiobiologiques ont démontré une réduction remarquable des dommages aux tissus normaux apportée par la pMBRT par rapport à la protonthérapie (PT) standard.
Dans un premier temps, une viabilité cellulaire réduite et une réponse inflammatoire accrue ont été observées après une irradiation homogène à faisceau large, par rapport à l’irradiation pMBRT, dans un modèle tridimensionnel (3D) de peau humaine artificielle [20]. De plus, des oreilles de souris ont été irradiées soit avec des mini-faisceaux de protons, soit par une irradiation de PT standard. La même dose déposée (60 Gy) a entraîné un degré d’œdème, d’érythème ou de desquamation significativement plus élevé chez les animaux ayant reçu une irradiation à faisceau large (PT standard) que chez ceux ayant reçu une irradiation pMBRT [19].
Une autre expérience pionnière a permis de démontrer la remarquable réduction de la neurotoxicité apportée par la pMBRT [22]. Des cerveaux entiers (à l’exception du bulbe olfactif) de deux groupes (n = 8/chacun) de rats normaux (âgés de 7 semaines) ont été irradiés: (i) le premier groupe a reçu une irradiation aux protons standard, avec des doses élevées (25 Gy en une session); (ii) le second groupe a reçu une irradiation pMBRT avec des doses maximales de 58 Gy par fraction (correspondant à la même dose moyenne que dans le groupe (i) de 25 Gy). Le suivi a duré 6 mois, pendant lesquels une étude longitudinale d’imagerie par résonance magnétique (IRM) à l’aide d’un scanner IRM 7-T pour petits animaux, ainsi que des analyses histologiques et immunochimiques, ont été réalisées. Les rats traités par irradiation conventionnelle aux protons ont présenté des lésions cutanées de grade 3 (desquamation humide) et des lésions cérébrales étendues, notamment des nécroses, démyélinisation, astrogliose et activation microgliale. Au contraire, le traitement par pMBRT n’a entraîné aucun dommage significatif [22] (Figure 3, à gauche). Il a également été démontré que la pMBRT n’a pas d’impact significatif sur les processus cognitifs, moteurs ou émotionnels après des irradiations du cerveau entier de rat [21].
Cette réduction de la neurotoxicité observée avec la pMBRT par rapport à la RT standard peut améliorer l’index thérapeutique dans les cas qui présentent de bons taux de contrôle de la tumeur mais qui s’accompagnent d’effets secondaires importants, comme l’astrocytome pédiatrique. Elle ouvre également la porte à un traitement plus efficace des tumeurs radiorésistantes, comme les gliomes de haut grade (GBM), qui restent l’un des diagnostics les plus difficiles en oncologie clinique.
En effet, dans une étude précédente sur des rats porteurs de gliomes, la pMBRT a montré une efficacité de contrôle de la tumeur égale ou supérieure à celle de la RT standard, et sans les effets secondaires observés dans différents modèles de gliomes de rat, ouvrant ainsi la possibilité de schémas d’irradiation plus agressifs [23, 24, 30].
En outre, dans certaines configurations, un taux de survie à long terme de 70% ( >6 mois, sans tumeur) a été obtenu dans le groupe pMBRT [24]. Voir Figure 3, à droite. La durée moyenne de survie était nettement supérieure à celle du groupe PT standard, malgré la couverture hétérogène de la dose cible. En outre, la stérilisation de la tumeur a même été obtenue dans les cas où l’espacement des faisceaux était important (3,5 mm) et où de grandes zones de la tumeur ont reçu des doses faibles (non tumoricides) [30]. Cela contredit le paradigme classique de la RT qui exige le dépôt d’une dose létale dans chaque cellule tumorale pour l’ablation de la tumeur. De plus, le fait que de grandes zones (voire le cerveau entier) puissent être irradiées sans effets secondaires significatifs permettrait de surmonter la nature infiltrante de la tumeur, l’une des principales difficultés à affronter dans le traitement des gliomes.
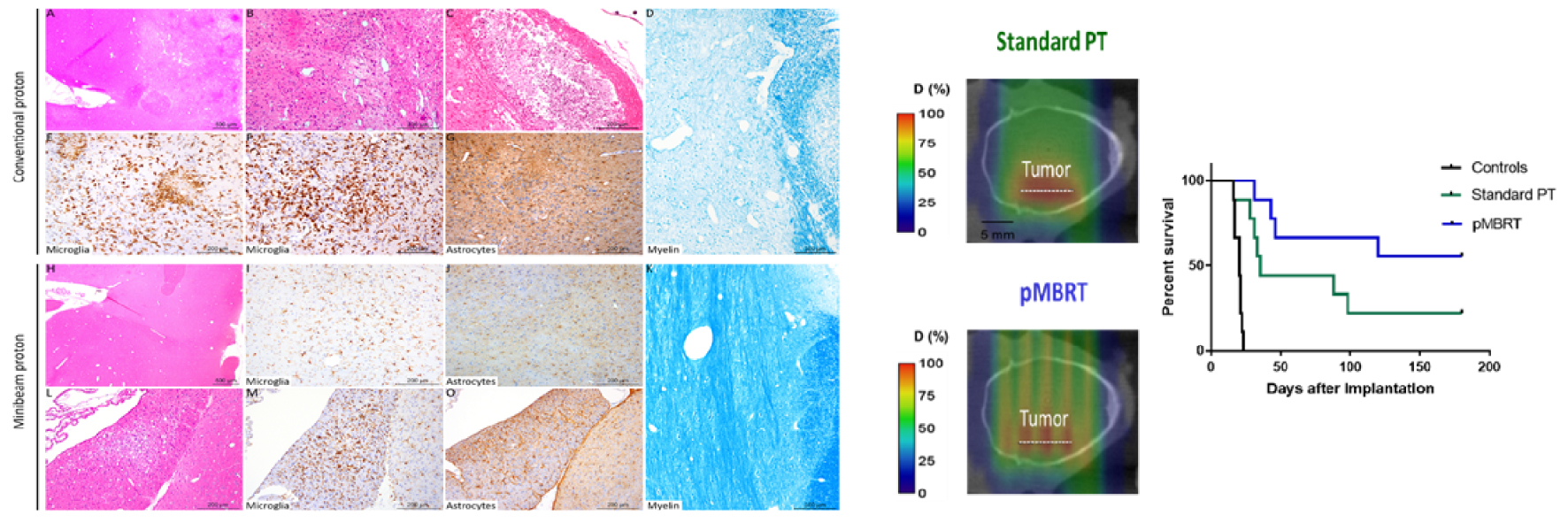
Une illustration du gain net de l’index thérapeutique de la pMBRT. A gauche : toxicité minimale de la pMBRT. Les analyses histopathologiques et immunohistochimiques ont révélé des dommages importants dans le groupe conventionnel (A–G). Irradiation conventionnelle : (A) Lésions multifocales à coalescentes caractérisées par (B) un œdème, une nécrose et une gliose. (C) Lésions plus graves avec cavitation et minéralisation. (D) Une destruction de la myéline a également été observée. (E, F) Activation microgliale et nodules (microgliose). (G) Activation des astrocytes avec une augmentation marquée de l’immunomarquage GFAP (astrogliose). Irradiation par mini-faisceaux : (H) Aucune lésion n’a été observée chez la plupart des rats (7 sur 8), avec (I, J) des réseaux microgliaux et astrocytaires normaux, et (K) une organisation normale de la myéline. Pour un seul rat : (L) Une inflitration microscopique et une légère destruction du neuropil ont été observées, associées à une microgliose (M) et une astrogliose (O) microscopiques focales. Tiré de [22]. Centre : Distribution des doses reçues par les rats porteurs de tumeurs. A droite : Contrôle de la tumeur. Courbes de survie des rats porteurs de tumeurs : témoins (ligne noire) ; PT standard (vert) ; pMBRT avec dose quasi-homogène dans la cible (bleu). On observe une augmentation de la durée de vie. Tiré de [24]. Masquer
Une illustration du gain net de l’index thérapeutique de la pMBRT. A gauche : toxicité minimale de la pMBRT. Les analyses histopathologiques et immunohistochimiques ont révélé des dommages importants dans le groupe conventionnel (A–G). Irradiation conventionnelle : (A) Lésions multifocales ... Lire la suite
Les études menées à ce jour ont utilisé un schéma d’irradiation à fractionnement temporel unique. La RT standard utilise généralement un fractionnement temporel de la dose pour profiter à la fois de la réoxygénation de la tumeur et de la redistribution des cellules vers des phases plus sensibles du cycle cellulaire (fin de la phase G2 et phase M) [31]. La toxicité locale des tissus est également réduite en raison de l’augmentation du temps disponible pour la réparation des dommages sublétaux, en plus du repeuplement des fractions cellulaires [31]. Ainsi, le fractionnement temporel pourrait également contribuer à l’avantage inhérent du fractionnement spatial de la dose pour la préservation des tissus normaux. Une étude récente a rapporté, pour la première fois, la réponse de rats porteurs d’un gliome à un fractionnement temporel de la pMBRT délivrée sous une géométrie de faisceaux entrelacés. Une augmentation significative de la durée médiane de survie a été obtenue lorsque la dose était délivrée sur deux séances plutôt qu’en une seule fraction. En outre, 83% des survivants à long terme présentaient une absence totale de cellules tumorales, ce qui est 3,3 fois supérieur à ce qui est observé avec une irradiation conventionnelle à large faisceau, sans excès de toxicité. Ce résultat pourrait faciliter la voie vers des traitements par pMBRT.
Par rapport aux dommages directs causés par les rayonnements ionisants, le pMBRT semble activer des mécanismes biologiques différents, le système immunitaire jouant déjà un rôle important [32]. D’autres facteurs peuvent inclure des effets de signalisation cellulaire, c’est-à-dire des effets de type « bystander » [33], et l’effet préférentiel sur la vascularisation tumorale par rapport à la vascularisation normale [4]. La participation de ces phénomènes doit être étudiée de manière plus approfondie avec une approche globale. En outre, la manière dont ces effets se traduisent lorsque la distribution spatiale de la dose est modifiée n’est toujours pas claire. En effet, aucune évaluation systématique de l’influence de la largeur et de l’espacement des faisceaux sur la réponse tissulaire à la RT fractionnée spatialement n’a été réalisée.
3. Perspectives : vers les essais cliniques
L’objectif ultime est de traduire et de reproduire en clinique les résultats de la pMBRT issus des expériences sur les petits animaux.
En effet, dix patients atteints de tumeurs de grande taille ont déjà été traités par protonthérapie GRID (faisceaux de 1 cm de large) [34] dans un but palliatif. Ces patients avaient des tumeurs qui ne pouvaient pas être traitées avec des photons, une irradiation standard à large faisceau ou une GRID aux photons en raison de la tolérance des tissus normaux. Le taux d’amélioration en termes de soulagement des symptômes et de régression partielle de la tumeur était de 80% pour tous les patients, tandis que des effets secondaires aigus (grade 1/2) sont apparus chez 50% des patients, qui les ont tous bien tolérés.
Les données expérimentales recueillies jusqu’à présent indiquent que la pMBRT peut augmenter l’index thérapeutique, permettant de passer d’un traitement palliatif à un traitement curatif. Les patients qui devraient bénéficier le plus des avantages de la pMBRT sont ceux qui souffrent de lymphomes, de gliomes de bas grade ou de lésions bénignes, comme les méningiomes ; ces patients peuvent profiter de la réduction des effets secondaires tout en conservant un contrôle local comparable des tissus malades. De plus, chez les patients atteints de tumeurs résistantes aux radiations, comme les GBM, les ostéosarcomes, les chordomes ou les chondrosarcomes, la pMBRT peut faciliter l’administration de doses potentiellement curatives plus élevées dans la tumeur. Les premiers essais thérapeutiques pourraient porter sur des tumeurs cérébrales primitives de mauvais pronostic, comme les gliomes récurrents.
La première étude théorique a été menée sur les plans de traitement possibles de deux tumeurs cérébrales, le gliome et le méningiome, chez des patients virtuels dérivés de dossiers d’imagerie anonymes de patients humains. Les plans de traitement pMBRT ont été comparés à des plans de protonthérapie standard, et ils ont satisfait aux critères recommandés pour des histogrammes dose-volume cliniquement acceptables dans les organes à risque. Une homogénéité satisfaisante a été obtenue dans la cible, tandis qu’un niveau similaire de modulation spatiale de la dose a été obtenu dans les tissus normaux, comme cela a été observé dans les études précliniques [35]. Les recherches futures se concentreront sur l’élucidation des mécanismes sous-jacents, sur la manière dont ces effets se traduisent lorsque la distribution spatiale est modifiée et, en lien avec cela, sur la détermination de la meilleure manière de prescrire la dose dans le cadre de la pMBRT, c’est-à-dire en termes de pic, de vallée, de dose moyenne ou, potentiellement, d’une nouvelle métrique.
4. Conclusion
La pMBRT est une nouvelle approche de thérapie anticancéreuse qui promet un indice thérapeutique élevé dans les cas difficiles à traiter, tels que les GBM. La pMBRT est considérée comme particulièrement intéressante pour une utilisation en oncologie pédiatrique en raison de sa neurotoxicité remarquablement faible. La pMBRT offre le meilleur équilibre entre les faisceaux larges employés dans la thérapie GRID et la thérapie en réseau, toutes deux actuellement limitées aux traitements palliatifs, et les conditions extrêmes de la radiothérapie par microfaisceaux qui rendent difficile le passage de cette dernière technique aux traitements des patients. L’alliance des protons et de la MBRT permet de combiner les avantages physiques des protons pour la thérapie avec les avantages biologiques de la MBRT, promettant un traitement optimal. En effet, il a été démontré que la forte modulation spatiale de la dose dans la pMBRT entraînait une réduction significative des effets secondaires tout en assurant un contrôle de la tumeur (gliome de haut grade) équivalent ou supérieur à celui de l’irradiation homogène standard dans des expériences sur de petits animaux. Malgré sa jeunesse, les données biologiques recueillies jusqu’à présent remettent en question le paradigme classique de la RT et encouragent la préparation d’essais cliniques de phase 1. Cette préparation est en cours à l’Institut Curie.
Conflit d’intérêt
Les auteurs n’ont aucun conflit d’intérêt à déclarer.
Remerciements
Y. Prezado remercie chaleureusement le travail des membres actuels et anciens de son équipe « Nouvelles approches en radiothérapie », le personnel du centre de protonthérapie d’Orsay et la plateforme de radiothérapie expérimentale de l’Institut Curie. Y. Prezado remercie le Conseil européen de la recherche (ERC) pour son financement dans le cadre du programme de recherche et d’innovation Horizon 2020 de l’Union européenne (convention n° 817908).