1. Cortical tension in plants is of osmotic origin
Beyond their biochemical nature, living organisms can also be considered as physical objects experiencing mechanical stress. Because plants are usually fixed in the soil, they are exposed to external mechanical perturbations: gravity, wind, rain impacts, contact with neighboring living organisms (e.g. trees in a forest) and mineral elements (e.g. in the soil), water potential fluctuations (e.g. drought). Although these factors are essential to understand plant biology, here we analyze the internal cause of mechanical stress and its instructive role in morphogenesis.
Even in stable and controlled environmental conditions, plants constantly experience mechanical stress. The cell wall is quite porous. Water is the most abundant element in primary cell walls, and the cell wall contains negligible amounts of osmolytes. This generates a difference in osmotic pressure between the apoplast and the protoplast (water tends to enter the cell). Turgor pressure is defined as a hydrostatic pressure exerted from the cell wall onto the protoplast; it counterbalances the cell’s osmotic pressure [1]. Contrary to most animal cells, plant cells are highly pressurized structures, displaying a turgor pressure in the hPa–MPa range. Their stiff cell walls (with an apparent elastic modulus also in the hPa–MPa range) resist to this pressure [2]. From a mechanical point of view, growth involves both water uptake (driven by a water potential difference) and cell wall yielding to turgor pressure [1]. This is recapitulated in the Lockhart’s equation, which mathematically formalized plant cell growth as:
2. The plasma membrane, as a biochemical interface
The plasma membrane is a physical boundary emerging from the self-association of amphiphilic lipid molecules. In addition to lipids (phospholipids and sterols), the plasma membrane also hosts proteins, and carbohydrates groups that are attached to certain lipids or proteins. Proteins have a primary role in the ability of the cell to interact with its local environment. Lipids, and in particular phospholipids, ensure both structural and signaling roles (Figure 1, [11]).
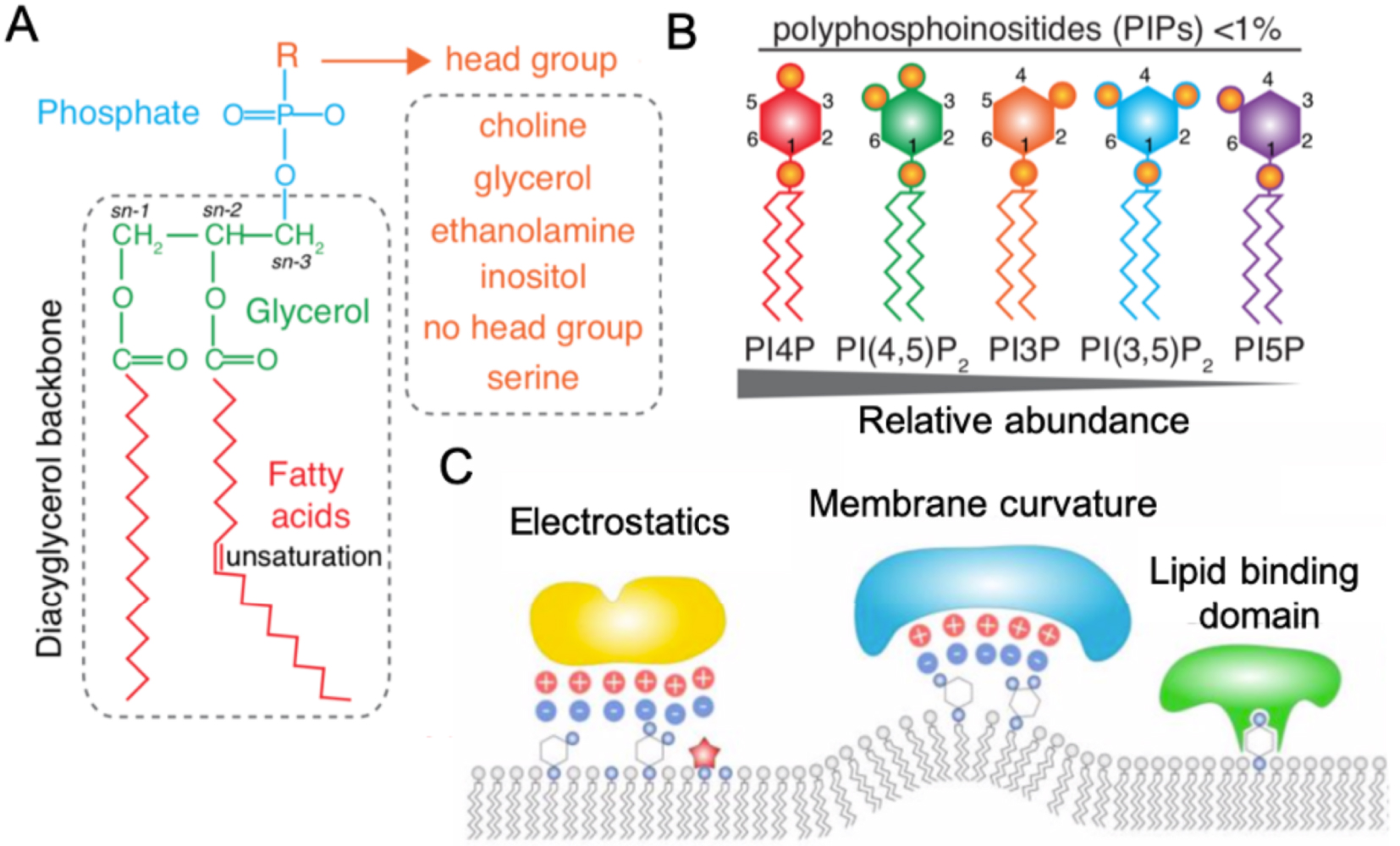
Phospholipid structure and properties. (A) Representation of a phospholipid structure depending of the head group, several phospholipids can be formed (orange). (B) Schematic representation of the detailed structure of the different phosphoinositide. Their relative abundance is indicated below. (C) Membrane targeting by anionic lipids. Representations of different scenario in which anionic lipids allow membrane targeting: through their electrostatics field (left), membrane curvature (middle) and directly through a lipid binding domain specifically recognized by proteins (right). Reproduced from [11].
Structural phospholipids are abundant. This includes phosphatidylcholine (PC) and phosphatitdylserine (PS), which are essential to establish a hydrophobic bilayer. Although they are considered as structural lipids, recent evidences have highlighted that these lipids can accumulate and form patterns, which have consequences on cell behavior and thus, could act as signaling lipids too [12].
Signaling phospholipids are much less abundant. Moreover, these lipids have a fast turn-over and can be rapidly produced by kinases, phosphatases or phospholipases in an acute manner at their site of action, allowing the establishment of lipid gradients and patterns in cells and tissues, involved in plant development [11]. Signaling lipids include phosphatidic acid (PA) and phosphatidyl-inositol-phosphates (PIPs). PIPs are minor phospholipids, which can be phosphorylated on the 3rd, 4th and/or 5th position of the inositol head group to generate up to seven PIP species, such as phosphatidylinositol-4-phosphate (PI4P) and phosphatidylinositol-4,5-bisphosphate (PI(4,5)P2) (Figure 1B) [13]. Signaling lipids ensure their regulatory role thanks to several features. First, they are negatively charged and can display interaction domains (e.g. Pleckstrin Homology domain (PH) [14, 15], Phox homology domain (PX) [16]), which allow them to interact and to recruit proteins to the plasma membrane (Figure 1C). They can also be involved in membrane trafficking. Finally, their hydrolysis can generate precursors of secondary messengers amenable to activate signaling cascades.
Through its position at the interface of the cell wall and the cytosol and through its role as a host of many candidate mechanosensors, the plasma membrane is generally thought to be a key, yet indirect, player for the transduction of mechanical signals. The plasma membrane composition can also have more direct roles in signaling, notably regulating both cell trafficking [17] and many signaling cascades [11, 18]. Although many studies start to decipher the role of the plasma membrane in cell signaling, its contribution in mechanical signaling pathways remains largely unknown in plants.
3. The plasma membrane is under tension
The plasma membrane is thought to be under tension because of the high turgor pressure in plant cells. Empirical evidence for the existence of membrane tension can be provided by the observation of protoplasts: in absence of a cell wall, protoplast appear spherical and explode if conditions are hypo-osmotic. Using micropipette aspiration, plasma membrane strength has been estimated in animal systems too [19]. Yet, in a tissue, with cell walls, one may wonder whether the plasma membrane truly experience tension.
The recent emergence of mechanosensitive probes enables to investigate how membrane tension affects biological process in vivo, and to reveal micro-mechanical stress patterns at the membrane levels. The development of such probes often relies on fluorescence lifetime imaging microscopy (FLIM) techniques, which measure the time a fluorophore remains in an excited state before emitting a photon. This lifetime directly depends on the microenvironment of the fluorochrome and thus, can provide crucial information on the molecular environment of the probe. In biological membranes, this fluorescent lifetime depends linearly on membrane tension within the cells, and thus, has been used to quantify tension in membranes.
In particular, Michels and colleagues, have designed four microviscosity probes, which function as molecular rotors, each one targeting a specific compartment of the cell (i.e., cell wall, plasma membrane, cytoplasm and vacuole) [20]. These boron-dipyrromethene (BODIPY)-based molecular rotors are fluorescent probes, characterized by a fluorescent lifetime and intensity, and which, upon photoexcitation, induce an internal rotation. The rotation rate can be estimated through the fluorescent lifetime and directly depend on the environment of the probe, thus providing information about the viscosity and the solvent polarity. For instance, lifetime quantifications for the cell wall probe would scale with the network mesh size, while lifetime quantification for the plasma membrane probe would scale with lipid spacing (and thus indirectly with lipid phase transition and tension). Using these probes, the authors managed to establish time-dependent micro-viscosity maps of multicellular tissues with a subcellular resolution, providing a new tool to address the role of mechanics in the regulation of biological events. Importantly, fluctuations in membrane tension could be validated experimentally: when cells in culture were exposed to hyperosmotic conditions, a reduction in the rotational motion (i.e. longer lifetime) of the probe could be recorded, consistent with induced compression. Conversely, in rapidly growing root hair where membrane tension is likely high, increased rotational motion (i.e. shoter lifetime) was recorded (Figure 2, [20]).
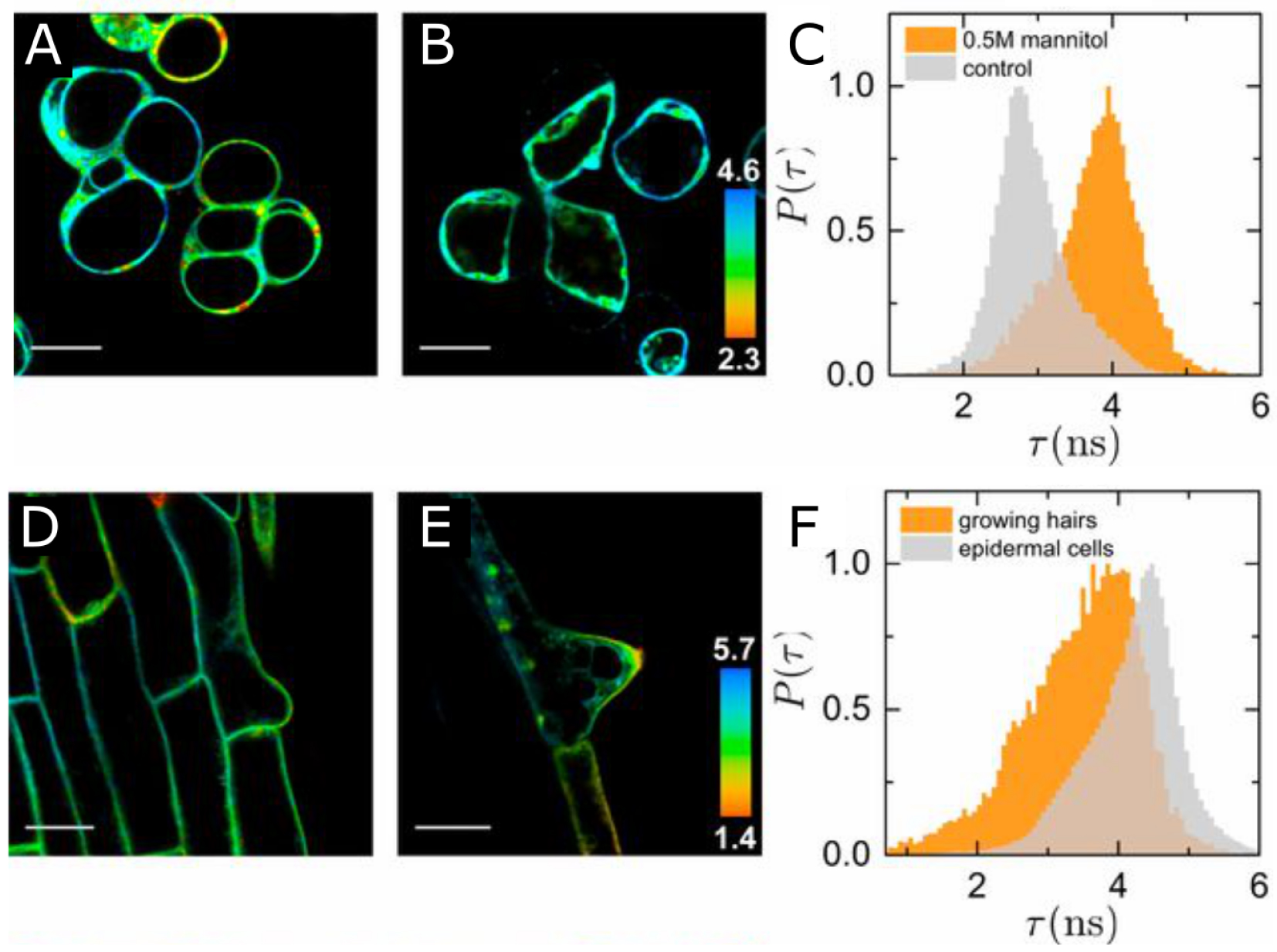
Probing membrane tension. (A–C) FLIM images of Arabidopsis suspension-cultured cells before (A) and after (B) osmotic shock with 0.5 M mannitol to reduce plasma membrane tension (and corresponding lifetime distributions for cells (C)). (D–F) FLIM images of growing root hairs in the maturation zone (D, E) and corresponding lifetime distributions (F) in regular epidermal cells and in growing root hairs for epidermal cells and root hairs. Scale bar: 20 μm. Reproduced from [20].
The recent development of the Fluorescent Lipid Tension Reporter (FliptR), also based on lifetime measurements, have also demonstrated its efficiency to measure membrane tension in animal cells, and to detect tension changes after osmotic treatment [21]. It remains to be tested in plant cells.
Globally, the use of mechanosensitive probes can reveal, in a non-invasive method, how plasma membrane tension is adaptively modified during dynamics events, like growth or cell division. In addition, these probes can be used in combination, e.g. with markers highlighting of plasma membrane microdomains, to provide critical information about the impact of membrane composition and/or ordering on plasma membrane mechanical behavior. Despite an increased interest for mechanobiology during the past two decades, the role of the plasma membrane composition/regulation in mechanotransduction largely remains an open question in plants [22].
4. Direct contributions of plasma membrane tension to cell functions
Membrane tension affects cell trafficking. Exocytosis is promoted when plasma membrane tension increases. For instance, hypoosmotic shocks or substrate stretching induce an increase of membrane tension, which has been correlated with an increase of exocytosis in animal cells [23]. Conversely, the use of ethanol and dimethyl sulfoxide (DMSO), which are both known to decrease the plasma membrane rigidity and tension, enhance endocytosis (Figure 3A) [24]. Such tension-dependent trafficking can impact proteins localization and recycling in plants too. For instance, several studies indicate that PIN1 protein localization is sensitive to membrane tension. Mechanical perturbations (e.g., osmotic treatments or tissue compression), leading to modification of membrane tension also affect the recruitment of the auxin efflux carrier PIN1 to the plasma membrane in tomato shoot apex [25]. Consistent with these results, PIN1 polarity correlates with patterns of tensile stress in Arabidopsis shoot apical meristem (Figure 3B and C, [26].
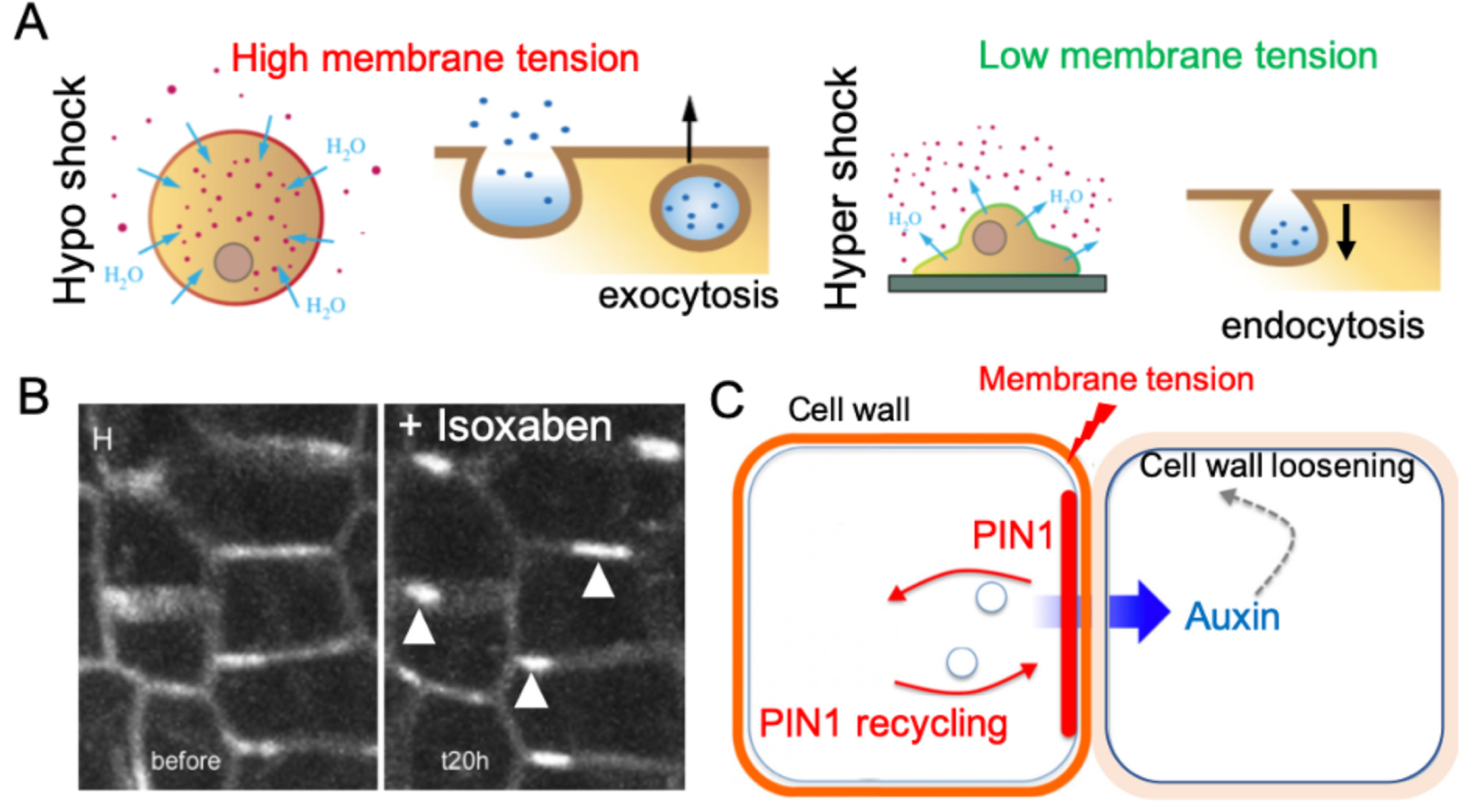
Membrane tension affects vesicle trafficking. (A) Hypoosmotic shock leads to cell swelling, which increases membrane tension and promotes exocytosis. Hyperosmotic shock induces a decrease in membrane tension and promotes endocytosis (adapted from [8]). (B) PIN1-GFP localization in plasma membrane before (left) and 20 h (right) after treatment with isoxaben, a cellulose synthase inhibitor which weakens the cell wall and arguably increase membrane tension. White arrowheads indicate increased PIN1 recruitment at the plasma membrane. Meristematic cells are ca. 5 μm wide (adapted from [52]). (C) A model proposing how membrane tension could trigger PIN1 polarity (adapted from [53]). Masquer
Membrane tension affects vesicle trafficking. (A) Hypoosmotic shock leads to cell swelling, which increases membrane tension and promotes exocytosis. Hyperosmotic shock induces a decrease in membrane tension and promotes endocytosis (adapted from [8]). (B) PIN1-GFP localization in plasma membrane ... Lire la suite
Furthermore, changes in membrane tension can impact the activity of proteins already present at the plasma membrane [27, 28]. Indeed, the application of tension to a membrane modifies its structure, e.g., reduces its thickness or curvature, that can in turn alter the energetic balance of proteins. This can lead to the stabilization of the open state of channels, as shown for MscL mechanosensitive channels [29]. More specifically, conformational changes of the channel result in membrane deformation that has a free energy cost. For instance, open channels have larger perimeter and thus induce a higher deformation in the surrounding membrane. However, concomitantly, opening of the channel results in a reduction in the free energy of the loading device. This model suggests that the equilibrium between two competing free energies determines which conformational state (e.g., opened or gated) is the most favorable [30]. Needless to say, such conformation changes, induced by modifications of the lipid environment, might also affect other mechanosensors, and in particular receptor-like kinases. The activity of aquaporins, which mediate most of the water transport across the plasma membrane [31], could also be affected by membrane tension, as shown for the human aquaporin AQP1 in Xenopus oocytes [32], feeding back on turgor pressure. This may even extend to plasmodesmata, which have recently been suggested to be mechanosensitive [33].
Last, modification of membrane tension directly impacts plasma membrane organization and order. This can lead to an increase of lipids orders in the case of tension, or conversely, to phosphoinositide clusters, membrane curvature or lipid packing defects under compression in animal fibroblasts (Figure 4A and B) [34]. Formation of lipid raft domains and/or modification of membrane curvature can be recognized by specific proteins (e.g. Bar-domain proteins), which can subsequently activate different signaling pathways. The contribution of membrane tension in protein clustering through changes in membrane curvature and/or composition has not been investigated in plants yet.
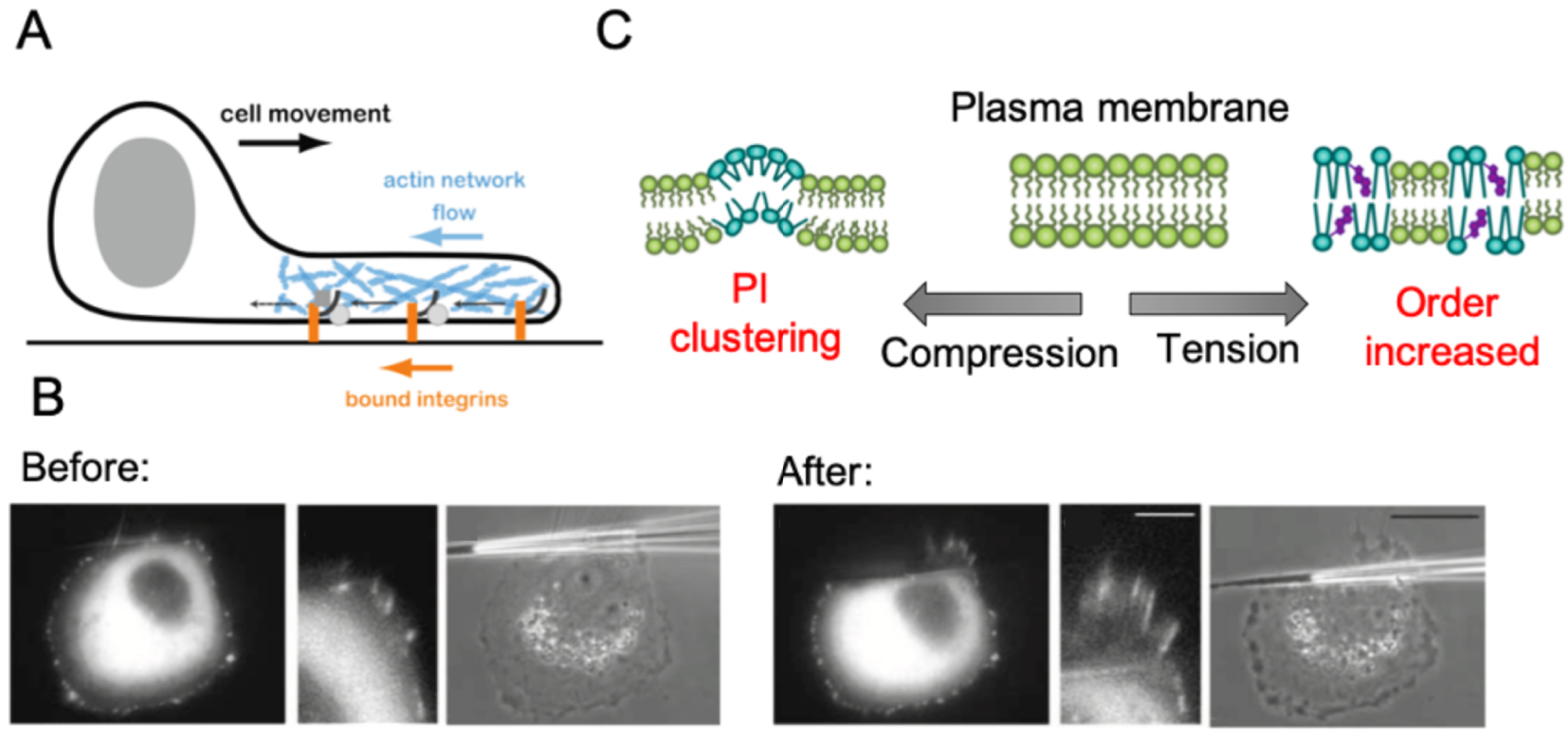
Membrane tension affects its own geometry and structure, as well as cytoplasmic factors. (A) Schematic representation of cell motility process in a fibroblast. Nascent adhesions form at the leading edge and attach to the substrate. Actin network growth and flow away from the leading edge leads to a rearward flux of adhesions. In particular, rearward flux is observed for bound integrins (adapted from [54]). (B) Vinculin marker (GFP) in transfected animal cells before and after the application of a pulling force produced by a micropipette shift. This reveals that pulling on the edge of a cell with a micropipette promotes the polymerization of F-actin (adapted from [55]). (C) Different responses to mechanical stimuli at the plasma membrane (adapted from [34]). Masquer
Membrane tension affects its own geometry and structure, as well as cytoplasmic factors. (A) Schematic representation of cell motility process in a fibroblast. Nascent adhesions form at the leading edge and attach to the substrate. Actin network growth and flow away ... Lire la suite
5. Integration of plasma membrane tension to cytosolic functions
Tension at the plasma membrane can impact the behavior of proteins that are attached at the cell cortex, and in particular, the cytoskeleton. This is most particularly the case for actin in animal systems (Figure 4C). By analogy, this likely impacts the dense network of cortical microtubules in plants. For instance, the Cellulose Synthase Interactive 1 (CSI1) protein physically binds the cellulose synthase protein (at the plasma membrane) and cortical microtubules (Figure 5). More generally, several proteins link microtubules to the lipid bilayer, and could play a role in signal transduction from the plasma membrane to the cytoskeleton, or vice-versa. Among them, CLIP-associating proteins (CLASPs) were shown to play an important role in microtubule network dynamics and organization in plant cells. Indeed, Clasp-1 mutants display a partial detachment of microtubules from the cortex, as shown by extensive waving, distortion and orientation changes of detached microtubules (Figure 5, [35]. Moreover, it has been demonstrated through live-imaging and computational modelling, that CLASP proteins, which accumulate at cell edges, overcome edge-induced microtubule depolymerization and enables microtubule growth around sharp edges. Overall, these data suggest that CLASP activity contribute to self-organization of cortical microtubules by governing microtubule-cortex attachment [35]. Further studies are required to investigate the role of plasma membrane composition and/or tension in CLASP functions.
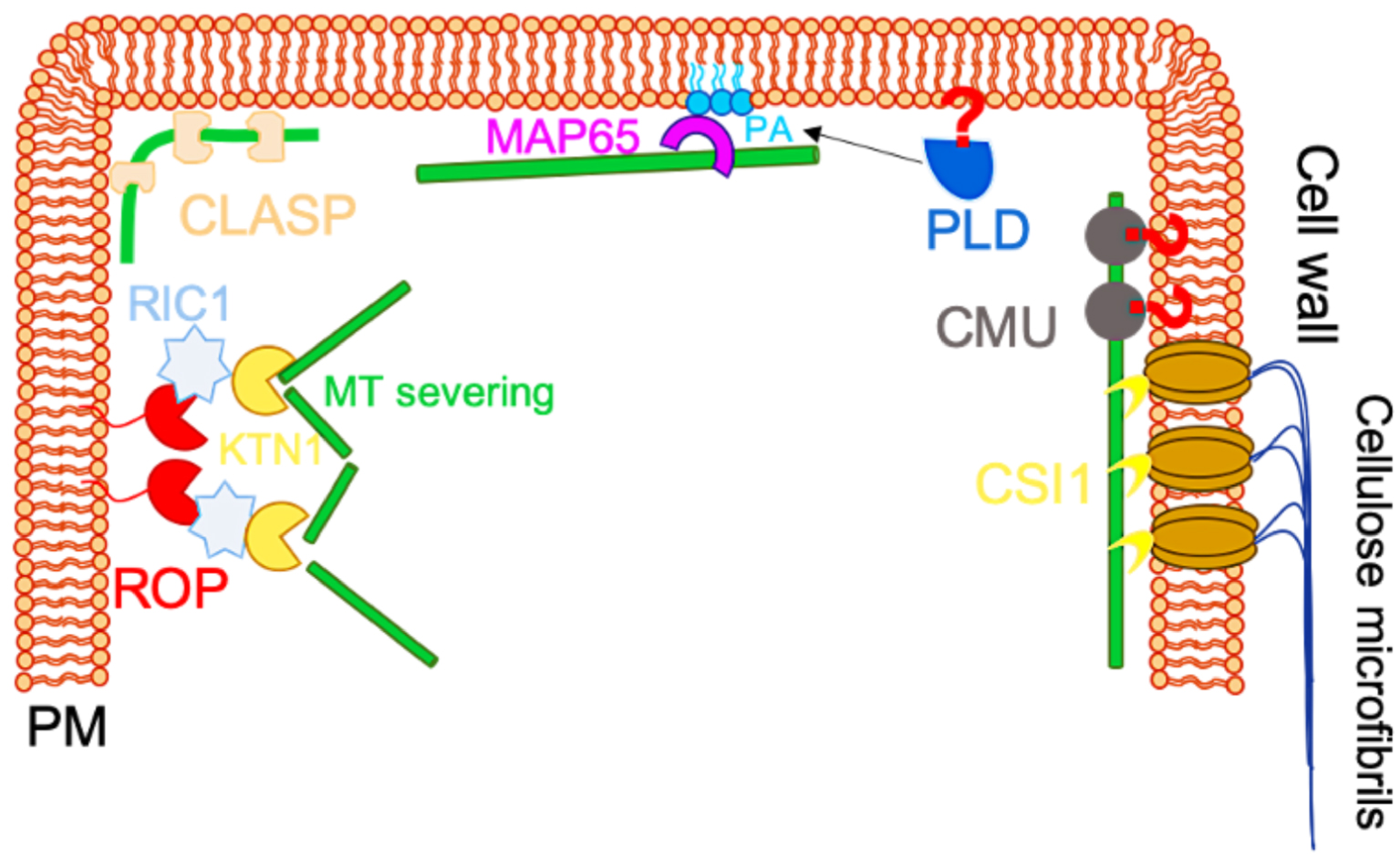
Schematic representation of proteins linking microtubule cytoskeleton to the plasma membrane. CSI1 directly connects cortical microtubules to the plasma membrane through the cellulose synthesis complex. Cellulose-microtubule proteins (CMUs) stabilize cortical microtubules at the cell cortex, preventing the dragging of cortical microtubules during cellulose synthesis. However, CMUs are not directly anchored to the PM. Therefore, a putative protein (indicated by a red question mark) could connect CMU and cortical microtubules to the plasma membrane. CLASP proteins are microtubule-associated proteins involved in the anchoring of CMTs to the cell cortex. Phospholipase D (PLD) has been proposed to anchor cortical microtubules to the PM and to contribute to cortical microtubule organization. PLD indirectly contributes to microtubule-plasma membrane anchoring by generating PA during salt stress, which, in turn, can activate MICROTUBULE-ASSOCIATED PROTEIN 65-1 (MAP65-1). ROPs have been involved in the organization of the cortical cytoskeleton, notably through the ROP6, RIC1 and katanin cacade (adapted from [22]). Masquer
Schematic representation of proteins linking microtubule cytoskeleton to the plasma membrane. CSI1 directly connects cortical microtubules to the plasma membrane through the cellulose synthesis complex. Cellulose-microtubule proteins (CMUs) stabilize cortical microtubules at the cell cortex, preventing the dragging of cortical ... Lire la suite
Interestingly, proteins involved in the regulation of plasma membrane composition have also been proposed to act as microtubules-plasma membrane proteins linkers. The most famous, and probably also the most debated, one is Phospholipase D (PLD), which is involved in PA synthesis (Figure 5). This idea is mainly supported by one study, which shows that a 90 kDa peptide (p90) in tobacco, sharing sequence similarity with Arabidopsis PLD𝛿, was associated with the plasma membrane and microtubules when transiently expressed in Bright Yellow 2 (BY-2) cells [36]. This association would be mediated through the PH/PX or C2 membrane association domain [37]. Other data suggest that PLD could indirectly mediate microtubule-plasma membrane via its product, PA. Indeed, PA can interact with MICROTUBULE-ASSOCIATED PROTEINS (MAP65-1), which is present in microtubule bundles. Consistently, decreased PA levels in a pld𝛼1 mutant results in microtubule depolymerization and salt sensitivity [38].
Rho-GTPases in plants (ROPs) may also play a key role to link cortical cytoskeleton and plasma membrane tension [39]. ROPs are specifically addressed to the plasma membrane by lipid-based post-translational modifications [39]. ROPs can both interact with the plasma membrane and other proteins. Two ROPs, ROP2 and ROP6, together with their interactors, ROP-interactive CRIB motif-containing protein1 (RIC1), have been identified to mediate microtubule-plasma membrane association [40]. RIC1 shows clear microtubule localization in pavement cells in vivo [41]. Both in vitro and in vivo studies have shown that RIC physically interacts with KATANIN1 in Arabidopsis, promoting microtubule-severing [42]. The increased severing resulted in higher proportion of parallel microtubules and reduced circularity in Arabidopsis pavement cells [42]. Furthermore, it has been reported that ROP2 and ROP6 antagonistically regulate the binding of RIC1 to microtubules. Indeed, while activated ROP2 binds to RIC1 and locally depletes the cortical microtubules, active ROP6 seems to promote the association of RIC1 with cortical microtubules [41, 43]. These results illustrate that pavement cell shape is tightly controlled through a dynamic interplay of ROPs and their interactions with the plasma membrane and microtubules (Figure 5).
This list is not exhaustive, and other candidates have been identified as potential protein linker between microtubules and the plasma membrane in plants [44]. Besides its role of hydrophobic bilayer, the plasma membrane thus plays a crucial role in mechanotransduction. Studies in animal systems, and in particular, in fibroblasts in culture, have been instrumental to dissect the relevant membrane contributions in mechanotransduction. While these could represent a good inspiration for further studies in plants, a current limitation is the lack of single cell approaches in plants.
6. Prospect: single-cell approaches in plants
Arguably, the most famous single cell system in plants is tobacco BY-2 cells. These cells are robust, and have been used in a large collection of studies, including revealing the response of the cytoskeleton to mechanical signals. The analysis of cortical microtubule orientation in centrifuged protoplasts have notably revealed that cortical microtubules usually orient parallel to the centrifugal force vector [45]. Similar trend was observed in protoplasts embedded in agarose and then stretched, where cortical microtubule array and the axis of elongation were reoriented [46]. Using the same method, and assuming that cell division plane can be a proxy for interphasic cortical microtubule orientation, Lynch and Lintilhac explored the impact of compression on cell division, and highlighted that the cell division plane is determined by both geometrical and mechanical cues [47]. More recently, artificial scaffolds have been tested to allow BY-2 cells to adhere on a substrate and grow, which is an essential step if one wants to reconcile single cell approach to cell behavior in tissues [48].
When considering the role of membrane tension in mechanotransduction, protoplasts may prove very useful. A system amenable to test both the effect of cortical geometry and membrane tension on the microtubule organization was developed. Indeed, by confining protoplasts in rectangular microwells, the impact on membrane curvature also affects the tension pattern: following Laplace–Young law, the transverse axis, where curvature is higher, also experiences maximal tension. When modifying the osmotic conditions, one can pressurize or depressurize the protoplast and relate differences in membrane tension to cytoskeletal behavior. Using this system, cortical microtubules were shown to align with minimal curvature when protoplasts are depressurized (arguably following a minimum of energy derived from the microtubule persistence length, [49]), and with maximal curvature when protoplasts are pressurized [50]. How such behavior is integrated with the regulators of microtubule dynamics remains to be investigated [51]. This system may offer a simpler approach to investigate the contribution of the plasma membrane in mechanotransduction, very much like fibroblasts in culture for the animal kingdom.
Conflicts of interest
Authors have no conflict of interest to declare.
French version
1. La tension corticale chez les plantes est d’origine osmotique
Au-delà de leur nature biochimique, les organismes vivants peuvent également être considérés comme des objets physiques subissant des contraintes mécaniques. Comme les plantes sont généralement fixées dans le sol, elles sont exposées à des perturbations mécaniques externes : gravité, vent, impacts de la pluie, contact avec des organismes vivants voisins (par exemple, les arbres dans une forêt) et des éléments minéraux (par exemple, dans le sol), fluctuations du potentiel hydrique (par exemple, la sécheresse). Bien que ces facteurs soient essentiels pour comprendre la biologie des plantes, nous analysons ici la cause interne des contraintes mécaniques et leur rôle dans la morphogenèse.
Même dans des conditions environnementales stables et contrôlées, les plantes subissent constamment des contraintes mécaniques. La paroi cellulaire est très poreuse. L’eau est l’élément le plus abondant dans les parois cellulaires primaires, et la paroi cellulaire contient des quantités négligeables d’osmolytes. Cela génère une différence de pression osmotique entre l’apoplaste et le protoplaste (l’eau a tendance à entrer dans la cellule). La pression de turgescence est définie comme une pression hydrostatique exercée par la paroi cellulaire sur le protoplaste; elle contrebalance la pression osmotique de la cellule [1]. Contrairement à la plupart des cellules animales, les cellules végétales sont des structures hautement pressurisées, affichant une pression de turgescence de l’ordre du hPa–MPa. Leurs parois cellulaires rigides (dont le module élastique apparent est également de l’ordre du hPa–MPa) résistent à cette pression [2]. D’un point de vue mécanique, la croissance implique à la fois l’absorption d’eau (induite par une différence de potentiel hydrique) et la soumission de la paroi cellulaire à la pression de turgescence [1]. Ceci est récapitulé dans l’équation de Lockhart, qui a formalisé mathématiquement la croissance des cellules végétales comme suit :
2. La membrane plasmique, une interface biochimique
La membrane plasmique est une barrière physique issue de l’auto-association de molécules lipidiques amphiphiles. En plus des lipides (phospholipides et stérols), la membrane plasmique contient également des protéines, et des groupes glucidiques qui sont attachés à certains lipides ou protéines. Les protéines jouent un rôle primordial dans la capacité de la cellule à interagir avec son environnement local. Les lipides, et en particulier les phospholipides, assurent à la fois des rôles de structure et de signalisation (Figure 1, [11]).
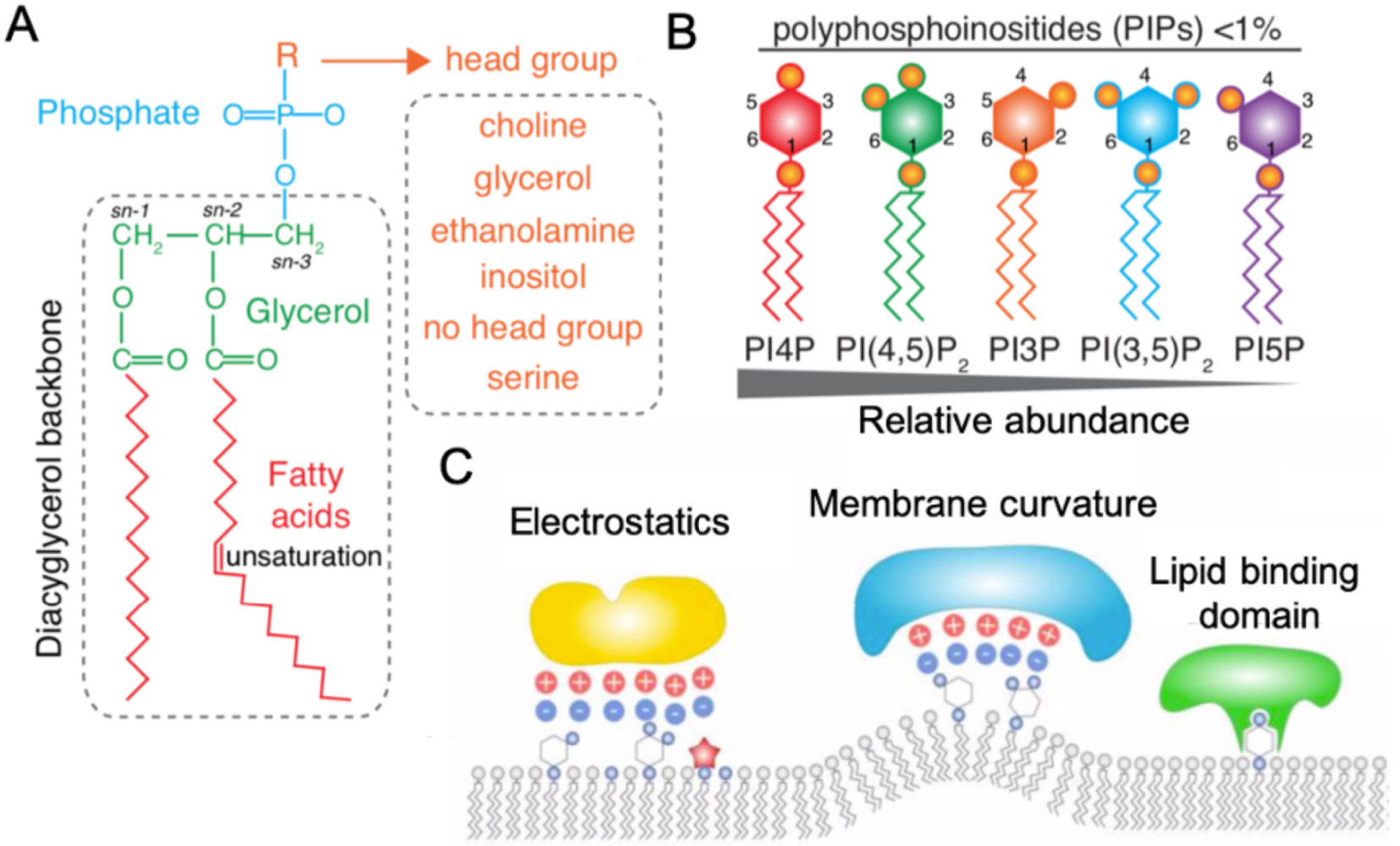
Structure et propriétés des phospholipides. (A) Représentation d’une structure phospholipidique. Selon le groupe de tête, plusieurs phospholipides peuvent être formés (orange). (B) Représentation schématique de la structure détaillée des différents phosphoinositides. Leur abondance relative est indiquée ci-dessous. (C) Ciblage membranaire par les lipides anioniques. Représentations des différents scénarios dans lesquels les lipides anioniques permettent le ciblage membranaire : par leur champ électrostatique (à gauche), la courbure de la membrane (au milieu) et directement par un domaine de liaison lipidique spécifiquement reconnu par les protéines (à droite). Reproduit à partir de [11]. Masquer
Structure et propriétés des phospholipides. (A) Représentation d’une structure phospholipidique. Selon le groupe de tête, plusieurs phospholipides peuvent être formés (orange). (B) Représentation schématique de la structure détaillée des différents phosphoinositides. Leur abondance relative est indiquée ci-dessous. (C) Ciblage membranaire ... Lire la suite
Les phospholipides structuraux sont abondants. Il s’agit notamment de la phosphatidylcholine (PC) et de la phosphatitdylsérine (PS), qui sont essentielles pour établir une bicouche hydrophobe. Bien qu’ils soient considérés comme des lipides structuraux, des résultats récents ont démontré que ces lipides peuvent s’accumuler et former des motifs avec des conséquences sur le comportement cellulaire. Ils pourraient donc également agir comme des lipides de signalisation [12].
Les phospholipides de signalisation sont beaucoup moins abondants. De plus, ces lipides ont un turn-over rapide et peuvent être rapidement produits par des kinases, des phosphatases ou des phospholipases de manière précise sur leur site d’action, permettant l’établissement de gradients lipidiques et de motifs dans les cellules et les tissus, impliqués dans le développement des plantes [11]. Les lipides de signalisation comprennent l’acide phosphatidique (PA) et les phosphatidyl-inositol-phosphates (PIP). Les PIP sont des phospholipides mineurs, qui peuvent être phosphorylés sur la 3ème, 4ème et/ou 5ème position du groupe inositol pour générer jusqu’à sept espèces de PIP, comme le phosphatidylinositol-4-phosphate (PI4P) et le phosphatidylinositol-4,5-bisphosphate (PI(4,5)P2) (Figure 1B) [13]. Les lipides de signalisation assurent leur rôle régulateur grâce à plusieurs caractéristiques. Tout d’abord, ils sont chargés négativement et peuvent présenter des domaines d’interaction (par exemple le domaine d’homologie Pleckstrin (PH) [14, 15], le domaine d’homologie Phox (PX) [16]), qui leur permettent d’interagir et de recruter des protéines à la membrane plasmique (Figure 1C). Ils peuvent également être impliqués dans le trafic membranaire. Enfin, leur hydrolyse peut générer des précurseurs de messagers secondaires susceptibles d’activer des cascades de signalisation.
Par sa position à l’interface de la paroi cellulaire et du cytosol et par son rôle d’hôte de nombreux candidats mécanosenseurs, la membrane plasmique est généralement considérée comme un acteur clé, mais indirect, de la transduction des signaux mécaniques. La composition de la membrane plasmique peut également jouer un rôle plus direct dans la signalisation, notamment en régulant le trafic cellulaire [17] et de nombreuses cascades de signalisation [11, 18]. Bien que de nombreuses études commencent à décrypter le rôle de la membrane plasmique dans la signalisation cellulaire, sa contribution dans les voies de signalisation mécanique reste largement inconnue chez les plantes.
3. La membrane plasmique est sous tension
On pense que la membrane plasmique est sous tension en raison de la pression de turgescence élevée dans les cellules végétales. Des preuves empiriques de la tension membranaire peuvent être fournies par l’observation de protoplastes : en l’absence de paroi cellulaire, les protoplastes apparaissent sphériques et explosent si les conditions sont hypo-osmotiques. En utilisant l’aspiration par micropipette, la force de la membrane plasmique a également été estimée dans des systèmes animaux [19]. Pourtant, dans un tissu, avec des parois cellulaires, on peut se demander si la membrane plasmique subit réellement une tension.

Sondage de la tension membranaire. (A–C) Images FLIM de cellules Arabidopsis cultivées en suspension avant (A) et après (B) un choc osmotique avec du mannitol 0,5 M pour réduire la tension de la membrane plasmique (et distributions de durée de vie correspondantes pour les cellules (C)). (D–F) Images FLIM de poils racinaires en croissance dans la zone de maturation (D, E) et distributions de durée de vie correspondantes (F) dans les cellules épidermiques régulières et dans les poils racinaires en croissance pour les cellules épidermiques et les poils racinaires. Barre d’échelle : 20 μm. Reproduit à partir de [20]. Masquer
Sondage de la tension membranaire. (A–C) Images FLIM de cellules Arabidopsis cultivées en suspension avant (A) et après (B) un choc osmotique avec du mannitol 0,5 M pour réduire la tension de la membrane plasmique (et distributions de durée de ... Lire la suite
L’émergence récente de sondes mécanosensibles permet d’étudier comment la tension de la membrane affecte les processus biologiques in vivo, et de révéler des modèles de contraintes micromécaniques au niveau de la membrane. Le développement de ces sondes s’appuie souvent sur les techniques de microscopie d’imagerie de la durée de fluorescence (FLIM), qui mesurent le temps pendant lequel un fluorophore reste dans un état excité avant d’émettre un photon. Cette durée de vie dépend directement du microenvironnement du fluorochrome et peut donc fournir des informations cruciales sur l’environnement moléculaire de la sonde. Dans les membranes biologiques, cette durée de vie de fluorescence dépend linéairement de la tension de la membrane à l’intérieur des cellules, et a donc été utilisée pour quantifier la tension dans les membranes.
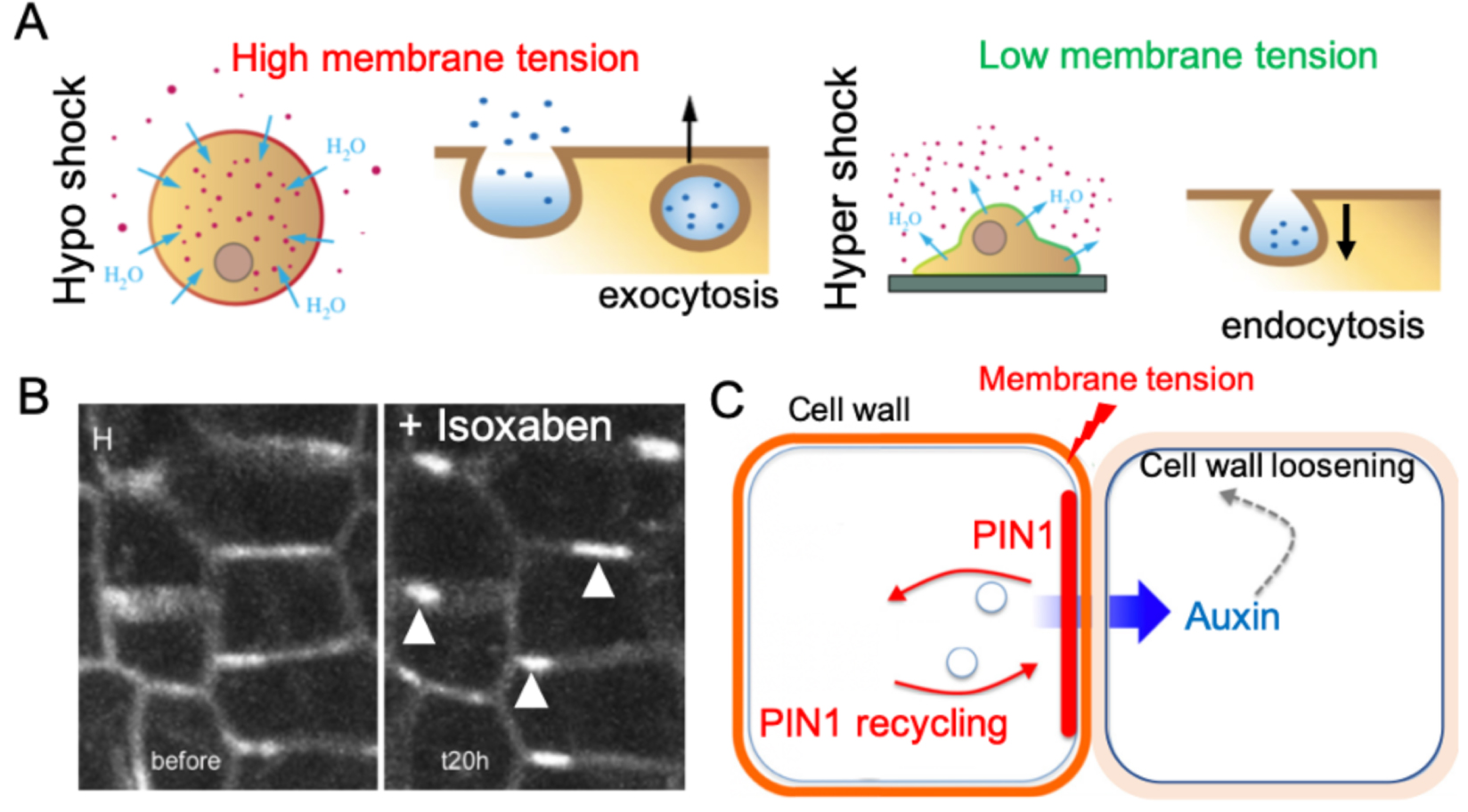
La tension membranaire affecte le trafic des vésicules. (A) Un choc hypoosmotique entraîne un gonflement des cellules, ce qui augmente la tension membranaire et favorise l’exocytose. Un choc hyperosmotique induit une diminution de la tension membranaire et favorise l’endocytose (adapté de [8]). (B) Localisation de PIN1-GFP dans la membrane plasmique avant (à gauche) et 20h (à droite) après traitement avec isoxaben, un inhibiteur de la cellulose synthase qui affaiblit la paroi cellulaire et augmente sans doute la tension membranaire. Les pointes de flèches blanches indiquent un recrutement accru de PIN1 au niveau de la membrane plasmique. Les cellules méristématiques ont une largeur d’environ 5 μm de large (adapté de [52]). (C) Un modèle proposant comment la tension membranaire pourrait déclencher la polarité de PIN1 (adapté de [53]). Masquer
La tension membranaire affecte le trafic des vésicules. (A) Un choc hypoosmotique entraîne un gonflement des cellules, ce qui augmente la tension membranaire et favorise l’exocytose. Un choc hyperosmotique induit une diminution de la tension membranaire et favorise l’endocytose (adapté ... Lire la suite
En particulier, Michels et ses collègues ont conçu quatre sondes de microviscosité, qui fonctionnent comme des rotors moléculaires, chacun ciblant un compartiment spécifique de la cellule (c’est-à-dire la paroi cellulaire, la membrane plasmique, le cytoplasme et la vacuole) [20]. Ces rotors moléculaires à base de bore-dipyrrométhène (BODIPY) sont des sondes fluorescentes, caractérisées par une durée de vie et une intensité fluorescentes, et qui, après photoexcitation, induisent une rotation interne. La vitesse de rotation peut être estimée par la durée de vie de la fluorescence et dépend directement de l’environnement de la sonde, fournissant ainsi des informations sur la viscosité et la polarité du solvant, par exemple. Par exemple, la quantification du temps de vie de la sonde de la paroi cellulaire dépendrait de la taille de la maille du réseau, tandis que la quantification du temps de vie de la sonde de la membrane plasmique dépendrait de l’espacement des lipides (et donc indirectement de la transition de phase et de la tension des lipides). Grâce à ces sondes, les auteurs ont réussi à établir des cartes de micro-viscosité en fonction du temps dans des tissus multicellulaires avec une résolution subcellulaire, fournissant ainsi un nouvel outil pour étudier le rôle de la mécanique dans la régulation des événements biologiques. Il est important de noter que les fluctuations de la tension membranaire ont pu être validées expérimentalement : lorsque les cellules en culture ont été exposées à des conditions hyperosmotiques, une réduction du mouvement de rotation (c’est-à-dire une durée de vie plus longue) de la sonde a pu être enregistrée, ce qui correspond à une compression induite. Inversement, dans le poil racinaire en croissance rapide où la tension membranaire est probablement élevée, un mouvement de rotation accru (c’est-à-dire une durée de vie plus courte) a été enregistré (Figure 2, [20]).
Le développement récent du Fluorescent Lipid Tension Reporter (FliptR), également basé sur des mesures de durée de vie, a également démontré son efficacité pour mesurer la tension membranaire dans les cellules animales, et pour détecter les changements de tension après un traitement osmotique [21]. Il reste à le tester dans des cellules végétales.
Globalement, l’utilisation de sondes mécanosensibles peut révéler, par une méthode non invasive, comment la tension de la membrane plasmique est modifiée de manière adaptative lors d’événements dynamiques, comme la croissance ou la division cellulaire. En outre, ces sondes peuvent être utilisées en combinaison, par exemple avec des marqueurs mettant en évidence les microdomaines de la membrane plasmique, pour fournir des informations essentielles sur l’impact de la composition et/ou de l’ordonnancement de la membrane sur le comportement mécanique de la membrane plasmique. Malgré un intérêt croissant pour la mécanobiologie au cours des deux dernières décennies, le rôle de la composition/régulation de la membrane plasmique dans la mécanotransduction reste largement une question ouverte chez les plantes [22].
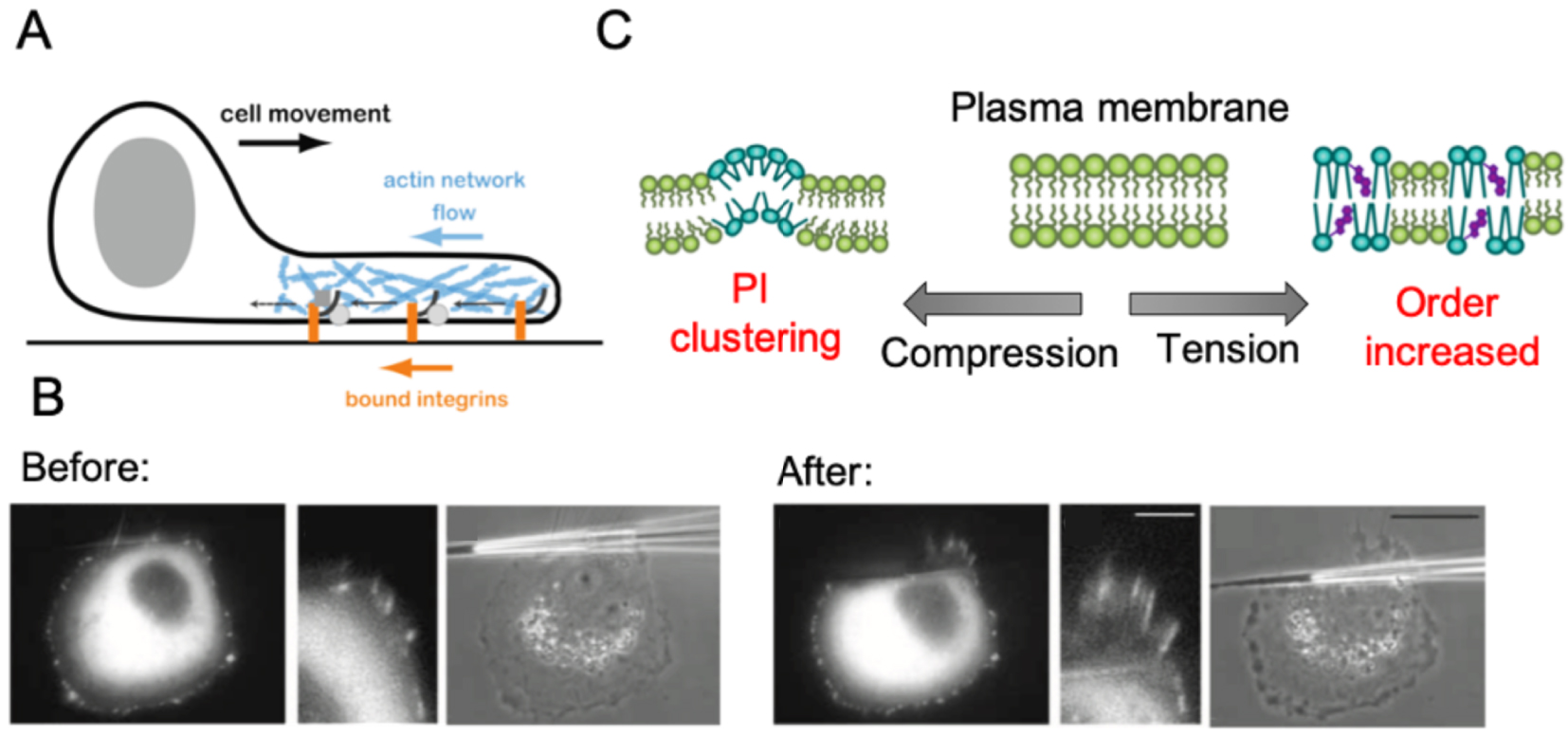
La tension membranaire affecte sa propre géométrie et structure, ainsi que des facteurs cytoplasmiques. (A) Représentation schématique du processus de motilité cellulaire dans un fibroblaste. Des adhésions naissantes se forment au niveau du bord d’attaque et s’attachent au substrat. La croissance et l’écoulement du réseau d’actine à partir du bord d’attaque entraînent un flux arrière d’adhésions. En particulier, le flux vers l’arrière est observé pour les intégrines liées (adapté de [54]). (B) Marqueur de la vinculine (GFP) dans des cellules animales transfectées avant et après l’application d’une force de traction produite par un déplacement de micropipette. Ceci révèle que le fait de tirer sur le bord d’une cellule avec une micropipette favorise la polymérisation de la F-actine (adapté de [55]). (C) Différentes réponses aux stimuli mécaniques au niveau de la membrane plasmique (adapté de [34]). Masquer
La tension membranaire affecte sa propre géométrie et structure, ainsi que des facteurs cytoplasmiques. (A) Représentation schématique du processus de motilité cellulaire dans un fibroblaste. Des adhésions naissantes se forment au niveau du bord d’attaque et s’attachent au substrat. La ... Lire la suite
4. Contributions directes de la tension de la membrane plasmique aux fonctions cellulaires
La tension de la membrane affecte le trafic cellulaire. L’exocytose est favorisée lorsque la tension de la membrane plasmique augmente. Par exemple, les chocs hypoosmotiques ou l’étirement du substrat induisent une augmentation de la tension membranaire, qui a été corrélée à une augmentation de l’exocytose dans les cellules animales [23]. À l’inverse, l’utilisation d’éthanol et de diméthylsulfoxyde (DMSO), qui sont tous deux connus pour diminuer la rigidité et la tension de la membrane plasmique, augmente l’endocytose (Figure 3A) [24]. Un tel trafic dépendant de la tension peut avoir un impact sur la localisation et le recyclage des protéines chez les plantes également. Par exemple, plusieurs études indiquent que la localisation de la protéine PIN1 est sensible à la tension de la membrane. Les perturbations mécaniques (par exemple, les traitements osmotiques ou la compression des tissus), entraînant une modification de la tension membranaire, affectent également le recrutement du transporteur d’efflux d’auxine PIN1 à la membrane plasmique dans l’apex des pousses de tomate [25]. En accord avec ces résultats, la polarité de PIN1 est corrélée avec les modèles de tension dans le méristème apical caulinaire d’Arabidopsis (Figure 3B et C, [26]).
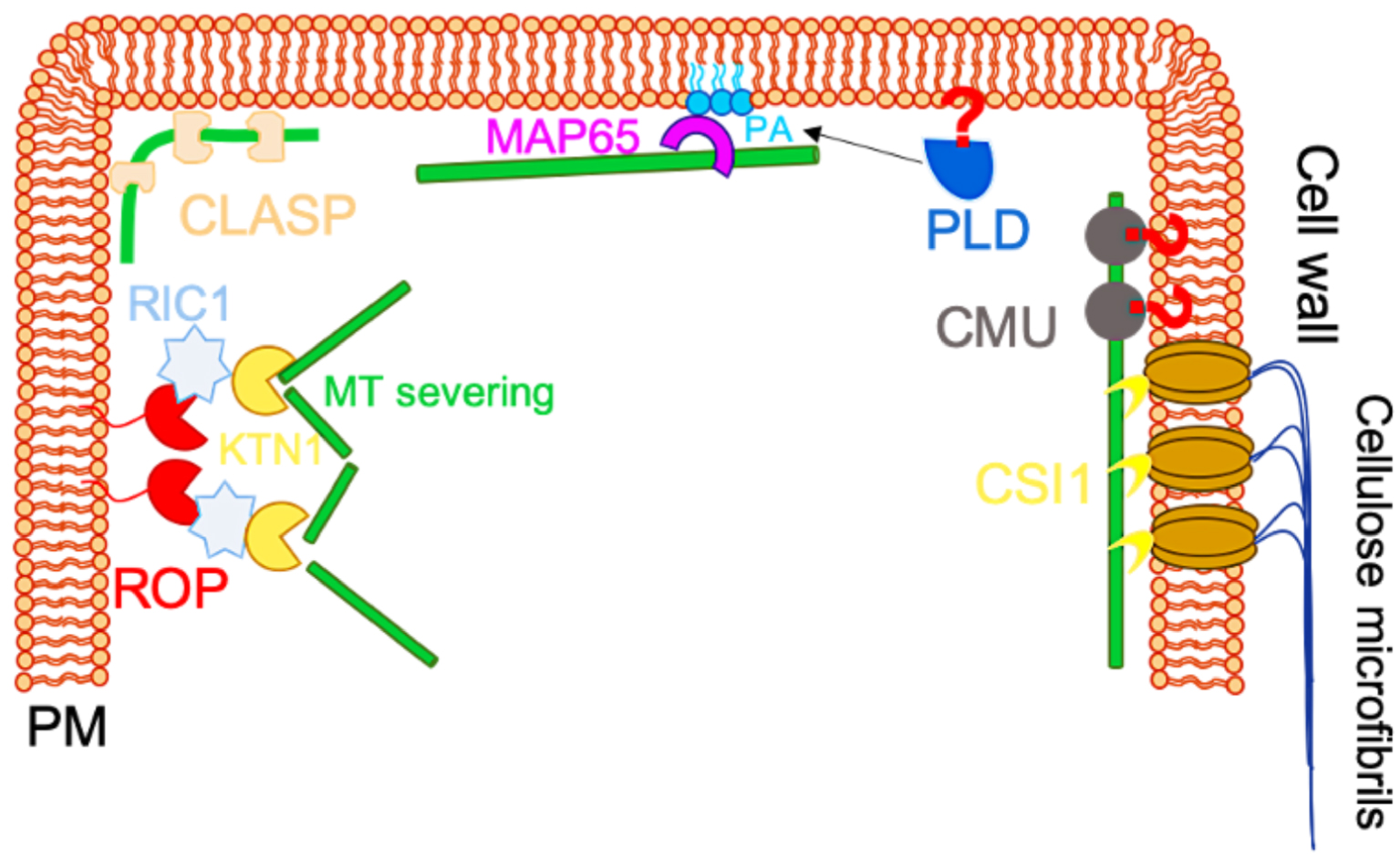
Représentation schématique des protéines reliant le cytosquelette des microtubules à la membrane plasmique. CSI1 relie directement les microtubules corticaux à la membrane plasmique par l’intermédiaire du complexe de synthèse de la cellulose. Les protéines cellulose-microtubules (CMU) stabilisent les microtubules corticaux au niveau du cortex cellulaire, empêchant l’entraînement des microtubules corticaux pendant la synthèse de la cellulose. Cependant, les CMUs ne sont pas directement ancrées à la PM. Par conséquent, une protéine putative (indiquée par un point d’interrogation rouge) pourrait connecter les CMU et les microtubules corticaux à la membrane plasmique. Les protéines CLASP sont des protéines associées aux microtubules impliquées dans l’ancrage des CMU au cortex cellulaire. La phospholipase D (PLD) a été proposée pour ancrer les microtubules corticaux à la PM et pour contribuer à l’organisation des microtubules corticaux. La PLD contribue indirectement à l’ancrage des microtubules à la membrane plasmique en générant du PA pendant le stress salin, qui, à son tour, peut activer la MICROTUBULE-ASSOCIATED PROTEIN 65-1 (MAP65-1). Les ROPs ont été impliqués dans l’organisation du cytosquelette cortical, notamment à travers la cacade ROP6, RIC1 et katanin (adapté de [22]). Masquer
Représentation schématique des protéines reliant le cytosquelette des microtubules à la membrane plasmique. CSI1 relie directement les microtubules corticaux à la membrane plasmique par l’intermédiaire du complexe de synthèse de la cellulose. Les protéines cellulose-microtubules (CMU) stabilisent les microtubules corticaux ... Lire la suite
De plus, les changements de tension membranaire peuvent avoir un impact sur l’activité des protéines déjà présentes au niveau de la membrane plasmique [27, 28]. En effet, l’application d’une tension à une membrane modifie sa structure, par exemple en réduisant son épaisseur ou sa courbure, ce qui peut à son tour modifier l’équilibre énergétique des protéines. Cela peut conduire à la stabilisation de l’état ouvert des canaux, comme cela a été montré pour les canaux mécanosensibles MscL [29]. Plus précisément, les changements conformationnels du canal entraînent une déformation de la membrane qui a un coût en énergie libre. Par exemple, les canaux ouverts ont un plus grand périmètre et induisent donc une plus grande déformation de la membrane environnante. Cependant, de manière concomitante, l’ouverture du canal entraîne une réduction de l’énergie libre de charge. Ce modèle suggère que l’équilibre entre deux énergies libres concurrentes détermine quel état conformationnel (par exemple, ouvert ou fermé) est le plus favorable [30]. Il va sans dire que de tels changements de conformation, induits par des modifications de l’environnement lipidique, pourraient également affecter d’autres mécanosenseurs, et en particulier les kinases de type récepteur. L’activité des aquaporines, qui assurent la plupart du transport de l’eau à travers la membrane plasmique [31], pourrait également être affectée par la tension membranaire, comme cela a été démontré pour l’aquaporine humaine AQP1 dans les ovocytes de Xenopus [32], en se nourrissant de la pression turgescente. Cela pourrait même s’étendre aux plasmodesmes, qui ont récemment été suggérés comme étant mécanosensibles [33].
Enfin, la modification de la tension membranaire a un impact direct sur l’organisation et l’ordre de la membrane plasmique. Cela peut conduire à une augmentation des ordres lipidiques en cas de tension, ou à l’inverse, à des amas de phosphoinositides, à une courbure membranaire ou à des défauts d’emballage lipidique sous compression dans les fibroblastes animaux (Figure 4A et B) [34]. La formation de domaines de radeaux lipidiques et/ou la modification de la courbure membranaire peuvent être reconnues par des protéines spécifiques (par exemple, les protéines à domaine Bar), qui peuvent ensuite activer différentes voies de signalisation. La contribution de la tension membranaire dans le regroupement des protéines par des modifications de la courbure et/ou de la composition de la membrane n’a pas encore été étudiée chez les plantes.
5. Intégration de la tension de la membrane plasmique aux fonctions cytosoliques
La tension au niveau de la membrane plasmique peut avoir un impact sur le comportement des protéines fixées au niveau du cortex cellulaire, et en particulier du cytosquelette. C’est plus particulièrement le cas de l’actine dans les systèmes animaux (Figure 4C). Par analogie, cela a probablement un impact sur le réseau dense de microtubules corticaux des plantes. Par exemple, la protéine Cellulose Synthase Interactive 1 (CSI1) lie physiquement la protéine cellulose synthase (à la membrane plasmique) et les microtubules corticaux (Figure 5). Plus généralement, plusieurs protéines relient les microtubules à la bicouche lipidique, et pourraient jouer un rôle dans la transduction du signal de la membrane plasmique au cytosquelette, ou vice-versa. Parmi elles, il a été démontré que les protéines CLASP jouent un rôle important dans la dynamique et l’organisation du réseau de microtubules dans les cellules végétales. En effet, les mutants clasp-1 présentent un détachement partiel des microtubules du cortex, comme le montrent l’ondulation, la distorsion et les changements d’orientation des microtubules détachés (Figure 5, [35]). De plus, il a été démontré par imagerie en direct et par modélisation informatique que les protéines CLASP, qui s’accumulent sur les coins des cellules, empêchent la dépolymérisation des microtubules induite par les coins et permettent donc la croissance courbe des microtubules dans ces coins. Dans l’ensemble, ces données suggèrent que l’activité de CLASP contribue à l’auto-organisation des microtubules corticaux en régissant la fixation des microtubules au cortex [35]. D’autres études sont nécessaires pour examiner le rôle de la composition et/ou de la tension de la membrane plasmique dans les fonctions de CLASP.
Des protéines impliquées dans la régulation de la composition de la membrane plasmique ont également été proposées pour lier microtubules corticaux et membrane plasmique. La plus connue, et probablement aussi la plus débattue, est la phospholipase D (PLD), qui est impliquée dans la synthèse des PA (Figure 5). Cette idée est principalement soutenue par une étude, qui montre qu’un peptide de 90 kDa (p90) du tabac, partageant une similarité de séquence avec la PLD𝛿 d’Arabidopsis, était associé à la membrane plasmique et aux microtubules lorsqu’il était exprimé de manière transitoire dans des cellules Bright Yellow 2 (BY-2) [36]. Cette association serait médiée par le domaine d’association membranaire PH/PX ou C2 [37]. D’autres données suggèrent que la PLD pourrait médier indirectement l’association microtubules-membrane plasmique via son produit, PA. En effet, PA peut interagir avec les MICROTUBULE-ASSOCIATED PROTEINS (MAP65-1), qui sont présentes dans les faisceaux de microtubules. De manière cohérente, la diminution des niveaux de PA chez un mutant pld𝛼1 entraîne une dépolymérisation des microtubules et une sensibilité au milieu salin [38].
Les Rho-GTPases dans les plantes (ROPs) peuvent également jouer un rôle clé pour relier le cytosquelette cortical et la tension de la membrane plasmique [39]. Les ROPs sont spécifiquement adressées à la membrane plasmique par des modifications post-traductionnelles à base de lipides [39]. Les ROPs peuvent à la fois interagir avec la membrane plasmique et avec d’autres protéines. Deux ROP, ROP2 et ROP6, ainsi que leur interacteur, la protéine ROP-interactive CRIB contenant un motif (RIC1), ont été identifiés comme médiateurs de l’association microtubules - membrane plasmique [40]. RIC1 montre une localisation claire des microtubules dans les cellules épidermiques pavimenteuses in vivo [41]. Des études in vitro et in vivo ont montré que RIC interagit physiquement avec KATANIN1 dans Arabidopsis, favorisant la séparation des microtubules [42]. L’augmentation de la séparation a entraîné une plus grande proportion de microtubules parallèles et une réduction de la circularité dans les cellules pavimenteuses d’Arabidopsis [42]. En outre, il a été signalé que ROP2 et ROP6 régulent de manière antagoniste la liaison de RIC1 aux microtubules. En effet, alors que la version active de ROP2 se lie à RIC1 et élimine les microtubules, la version active de ROP6 semble favoriser l’association de RIC1 avec les microtubules corticaux [41, 43]. Ces résultats illustrent le fait que la forme des cellules pavimenteuses est étroitement contrôlée par une interaction dynamique des ROPs et de leurs interactions avec la membrane plasmique et les microtubules (Figure 5).
Cette liste n’est pas exhaustive, et d’autres candidats ont été identifiés comme des protéines de liaison potentielles entre les microtubules et la membrane plasmique chez les plantes [44]. Outre son rôle de bicouche hydrophobe, la membrane plasmique joue donc un rôle crucial dans la mécanotransduction. Des études sur des systèmes animaux, et en particulier sur des fibroblastes en culture, ont permis de disséquer les contributions pertinentes de la membrane à la mécanotransduction. Bien qu’elles puissent constituer une bonne source d’inspiration pour d’autres études sur les plantes, l’absence d’approches unicellulaires chez les plantes constitue une limite actuelle.
6. Perspectives : approches unicellulaires chez les plantes
Le système unicellulaire le plus connu chez les plantes est sans doute celui des cellules BY-2 de tabac. Ces cellules sont robustes et ont été utilisées dans un grand nombre d’études, notamment pour révéler la réponse du cytosquelette aux signaux mécaniques. L’analyse de l’orientation des microtubules corticaux dans des protoplastes centrifugés a notamment révélé que les microtubules corticaux s’orientent généralement parallèlement au vecteur de la force centrifuge [45]. Une tendance similaire a été observée dans des protoplastes inclus dans de l’agarose puis étirés, où le réseau de microtubules corticaux et l’axe d’élongation ont été réorientés [46]. En utilisant la même méthode, et en supposant que le plan de division cellulaire peut être une approximation de l’orientation interphasique des microtubules corticaux, Lynch et Lintilhac ont exploré l’impact de la compression sur la division cellulaire, et ont souligné que le plan de division cellulaire est déterminé à la fois par des paramètres géométriques et mécaniques [47]. Plus récemment, des matrices artificielles ont été testées pour permettre aux cellules BY-2 d’adhérer à un substrat et de croître, ce qui est une étape essentielle si l’on veut concilier l’approche unicellulaire et le comportement des cellules dans les tissus [48].
Les protoplastes peuvent s’avérer très utiles pour étudier le rôle de la tension membranaire dans la mécanotransduction. Un système permettant de tester à la fois l’effet de la géométrie corticale et de la tension membranaire sur l’organisation des microtubules a été développé. En effet, en confinant les protoplastes dans des micropuits rectangulaires, l’impact sur la courbure de la membrane affecte également le modèle de tension : suivant la loi de Laplace–Young, l’axe transversal, où la courbure est plus élevée, subit également une tension maximale. En modifiant les conditions osmotiques, on peut pressuriser ou dépressuriser le protoplaste et relier les différences de tension de la membrane au comportement du cytosquelette. En utilisant ce système, il a été démontré que les microtubules corticaux s’alignent avec une courbure minimale lorsque les protoplastes sont dépressurisés (sans doute après un minimum d’énergie dérivée de la longueur de persistance des microtubules [49]), et avec une courbure maximale lorsque les protoplastes sont pressurisés [50]. La manière dont ce comportement est intégré aux régulateurs de la dynamique des microtubules reste à étudier [51]. Ce système peut offrir une approche plus simple pour étudier la contribution de la membrane plasmique dans la mécanotransduction, tout comme les fibroblastes en culture pour le règne animal.
Conflit d’intérêt
Les auteurs n’ont aucun conflit d’intérêt à déclarer.