1 Introduction
Salt accumulation in irrigated soils is one of the main factors that diminish crop productivity, since most of the plants are not halophytic. Excessive sodium (Na+) inhibits growth of many salt sensitive plants and glycophytes, which include most crop plants. The typical first response of all plants to salt stress is osmotic adjustment. Accumulation of compatible solutes in the cytoplasm is considered a mechanism contributing to salt tolerance [1]. To counter salt stress, plants increase the osmotic potential of their cells by synthesizing and accumulating compatible osmolytes such as proline (PRO) and glycine betaine (GB), which participates in osmotic adjustment [2]. Several plants, including halophytes, accumulate high PRO levels in response to osmotic stress as a tolerance mechanism to high salinity and water deficit [3,4]. In plants, PRO is synthesised from either glutamate or ornithine. However, the glutamate pathway is the primary route used under osmotic stress or nitrogen limitation conditions, whereas the ornithine pathway is prominent under high nitrogen input [5]. However, recent data suggest that glutamate is the major amino acid involved in PRO synthesis, since transgenic tobacco plants with reduced expression of cytosolic glutamine synthetase accumulated less PRO than non-transformed plants in response to salt stress [6]. Accumulation of PRO in plants under stress is a result of the reciprocal regulation of two pathways: [(-pyrroline-5-carboxylate synthetase (P5CS) and P5CR] and repressed activity of PRO degradation [5]. PRO catabolism is catalyzed by pyrroline-5-carboxylate dehydrogenase and PDH, a mitochondrial enzyme, whose activity had been shown to reduce during salt stress [7]. The first two steps of PRO biosynthesis are catalysed by P5CS by means of its γ-GK and glutamic-γ-semialdehyde dehydrogenase activities. Subsequently, the P5C formed is reduced by P5CR to PRO [8].
Salinity can cause hyperionic and hyperosmotic effects on plants, leading to membrane disorganization, increase in reactive oxygen species (ROS) levels and metabolic toxicity [9]. Generation of ROS cause rapid cell damage by triggering off a chain reaction [10]. In order to survive under stress conditions, plants are equipped with oxygen radical detoxifying enzymes such as superoxide dismutase (SOD), ascorbate peroxidase (APX), catalase (CAT), and antioxidant molecules like ascorbic acid (AA), α-tocopherol and reduced glutathione (GSH) [11]. ROS scavenging depends on the detoxification mechanism provided by an integrated system of non-enzymatically reduced molecules, like AA and glutathione, and enzymatic antioxidants [12]. Antioxidant mechanisms may provide a strategy to enhance salt tolerance in plants.
Oxidative stress is generally estimated in terms of lipid peroxidation (TBARS content) and H2O2 contents. H2O2 is an endogenous signalling molecule involved in plant responses to abiotic and biotic stresses such as extremes of temperature, light intensity, drought, pathogen, salinity, as well as stimuli such as plant hormones and gravity [7]. Accumulation of H2O2 will also lead to enhance the potential for production of hydroxyl radicals, which leads to lipid peroxidation (LPO) and membrane deterioration. Under saline environments, the plant lipid metabolism is interrupted as a result of oxidative damage to membrane lipids by active oxygen species (AOS) and LPO [13]. LPO can also be initiated enzymatically by lipoxygenases (LOX) [14] and this enzyme incorporates molecular oxygen into linoleic and linolenic acids, to form lipid hydroperoxides [15].
Though several countries in the world have a rich heritage of herbal drugs, very few can put claim for their procurement only from cultivated species. It is recently that some of these drugs have been subjected to systematic cultivation based on modern scientific information. Our reliance on wild sources of crude drugs and the lack of information of sound cultivation technology have resulted in gradual depletion of raw material from wild sources. Even though cultivation of medicinal plants offers a wide spectrum of advantages over their wild sources, it may be an uneconomical proposition for certain types of crude drugs that occur abundantly in their natural habitat [16]. More and more people are interested in the use of plants and plant-based drugs revived throughout the world, with increasing realization of health hazards and toxicity associated with the indiscriminate use of synthetic drugs and antibiotics [17]. In order to meet the ever-increasing demand of medicinal plants, for the indigenous systems of medicine as well as for the pharmaceutical industry, some medicinal plants need to be cultivated commercially, but the soil salinity and other forms of pollutions pose serious threats to plant production [18]. For the past several years, several scales of physiology have been applied to study responses to salt stress in field crops [3,4,19–21]. However, little information is gained about the physiological basis in terms of PRO metabolism and its effects on antioxidant potentials under salt stress in medicinal plants [1].
Pyllanthus amarus (Family: Euphorbiaceae), although considered as a problematic weed for farmers, is a valuable medicinal plant for herbalists [22]. The roots, leaves, fruits, milky juice, and whole plants are used in medicinal preparations. The bark yields a bitter principle called phyllanthin [23]. To the best of our knowledge, no information on the physiological response in terms of PRO and antioxidant metabolisms of P. amarus to NaCl application is available. The purpose of this study was to provide additional information on the oxidative damage (LPO and H2O2 contents), osmolyte concentration (GB and PRO contents), PRO metabolizing enzymes (γ-GK and PROX) and antioxidant enzymes (SOD, POX and CAT) activities in P. amarus under NaCl treatments.
2 Materials and methods
2.1 Plant materials and growth
The seeds of Phyllanthus amarus were obtained from the Herbal Folklore Research Centre, Andhra Pradesh, India. Seeds were then surface sterilized in an aqueous solution of 0.1% HgCl2 for 60 s to prevent fungal attack and rinsed in several changes of sterile water. The seeds were pre-soaked in 500 ml of deionized water (control) and 80 mM NaCl solutions for 12 h. Seeds were sown in plastic pots filled with soil mixture containing red soil, sand, and farmyard manure (FYM) at 1:1:1 ratio. Before sowing the seeds, the pots were irrigated with the respective treatment solutions and the electrical conductivity (EC) of the soil mixture was measured. Eight seeds were sown per pot and the pots were watered to the field capacity with deionized water up to 90 days after sowing (DAS); every care was taken to avoid leaching. The initial EC level of the soil was maintained by flushing each pot with the required volume of corresponding treatment solution on 45, 60, and 75 DAS. The position of each pot was randomized at 4-day intervals to minimize spatial effects in the greenhouse, where the temperature was 28 °C during the day and 22 °C at night; the relative humidity (RH) varied between 60 and 70%. The seedlings were thinned to one per pot on 20 DAS. Plants were uprooted randomly on 90 DAS and analysed for estimating the oxidative damage, osmolyte concentration, PRO, and antioxidant metabolisms.
2.2 Oxidative damage
2.2.1 H2O2 content
The hydrogen peroxide content was determined according to Velikova et al. [24]. 0.5 g of fresh plant material was homogenized in an ice bath with 5 ml of 0.1% (w/v) trichloro acetic acid (TCA). The homogenate was centrifuged at 12,000 rpm for 15 min and 0.5 ml of the supernatant was added to 0.5 ml of a 10 mM potassium phosphate buffer (pH 7.0) and to 1 ml of 1 M potassium iodide (KI). The absorbance of the supernatant was measured at 390 nm in a spectrophotometer (U-2001-Hitachi). The content of H2O2 was calculated by comparison with a standard calibration curve previously made by using different concentrations of H2O2.
2.2.2 Lipid peroxidation
LPO was studied for thiobarbituric acid reactive substances (TBARS) [25]. A fresh sample (0.5 g) was homogenized in 10 ml of 0.1% TCA, and the homogenate was centrifuged at 15,000 rpm for 15 min. To a 1.0-ml aliquot of the supernatant, 4.0 ml of 0.5% thiobarbituric acid (TBA) in 20% TCA were added. The mixture was heated at 95 °C for 30 min in the laboratory's electric oven, and then cooled in an ice bath. After centrifugation at 10,000 rpm for 10 min, the absorbance of the supernatant was recorded at 532 nm in a spectrophotometer. The TBARS content was calculated according to its extinction coefficient of 155 mM−1 cm−1 and expressed in units (U). One ‘U’ is defined as μmole of MDA formed min−1 mg−1 protein.
2.3 Osmolyte concentration
2.3.1 Proline content
The PRO content was estimated by the method of Bates et al. [26]. The plant material was homogenized in 3% aqueous sulfosalicylic acid and the homogenate was centrifuged at 10,000 rpm. The supernatant was used for the estimation of the PRO content. The reaction mixture consisted of 2 ml acid ninhydrin and 2 ml of glacial acetic acid, which was boiled at 100 °C for 1 h. After termination of reaction in an ice bath, the reaction mixture was extracted with 4 ml of toluene, and absorbance was read at 520 nm.
2.3.2 Glycine betaine content
The amount of GB was estimated according to the method of Grieve and Grattan [27]. The plant tissue was finely ground, mechanically shaken with 20 ml of deionized water for 24 h at 25 °C. The samples were then filtered and filtrates were diluted to 1:1 with 2 N H2SO4. Aliquots were kept in centrifuge tubes and cooled in ice water for 1 h. Cold KI–I2 reagent was added and the reactants were gently stirred with a vortex mixture. The tubes were stored at 4 °C for 16 h and then centrifuged at 10,000 rpm for 15 min at 0 °C. The supernatant was carefully aspirated with a fine glass tube. The periodide crystals were dissolved in 9 ml of 1,2-dichloroethane. After 2 h, the absorbance was measured at 365 nm using GB as standard.
2.4 Proline metabolizing enzymes
2.4.1 γ-Glutamyl kinase [ATP: l-glutamate 5-phosphotransferases (EC 2.7.2.11)] activity
γ-GK activity was assayed by the method of Hayzer and Leisinger [28]. Plant samples (1 g) were extracted with a 50 mM Tris-HCl buffer and centrifuged at 40,000 g for 30 min at 4 °C. 0.1 ml of reaction buffer was prepared by adding 0.1 ml 10 × ATP and 1.8 ml of extract; after incubated at 37 °C for 30 min, 2 ml of stop buffer were added. γ-GK activity was measured at 535 nm and expressed in units (U mg−1 protein). One unit of enzyme activity is defined as μg of γ-glutamylhydroxamate formed min−1 mg−1 protein.
2.4.2 Proline oxidase [L. Proline: O2 Oxidoreductase (EC 1.4.3.1)] activity
PROX activity was determined according to the method outlined by Huang and Cavalieri [29]. Plant samples (1 g) were extracted with 5 ml of Tris-HCl buffer (pH 8.5) grinding medium and centrifuged at 10,000 g for 10 min at 4 °C. The supernatant was again centrifuged at 25,000 g during 20 min at 4 °C. Three millilitres of assay mixture were prepared by taking 0.1 ml of extract, 1.2 ml of 50 mM Tris-HCl buffer (pH 8.5), 1.2 ml of 5 mM MgCl2, 0.1 ml of 0.5 mM NADP, 0.1 ml of 1 mM KCN, 0.1 ml of 1 mM phenazine methosulphate (PMS), 0.1 ml of 0.06 mM 2,6-dichlorophenol indophenol (DCPIP), and 0.1 ml of distilled water instead of PRO. The reaction was monitored at 600 nm at 25 °C using PRO to initiate the reaction; the increase of the OD value was noted at 0, 1, 2, 3, 4 and 5 min. PROX activity was expressed in U mg−1 protein (one U = mM DCPIP reduced min−1 mg−1 protein).
2.5 Antioxidant enzyme extractions and assays
2.5.1 Superoxide dismutase (SOD, EC 1.15.1.1)
The activity of SOD was assayed as described by Beauchamp and Fridovich [30]. The reaction mixture contained 1.17 × 10−6 M riboflavin, 0.1 M methionine, 2 × 10−5 M KCN and 5.6 × 10−5 M nitroblue tetrazolium (NBT) salt dissolved in 3 ml of 0.05 M sodium phosphate buffer (pH 7.8). Three millilitres of the reaction medium were added to 1 ml of enzyme extract. The mixtures were illuminated in glass test tubes by two sets of Philips 40-W fluorescent tubes in a single row. Illumination was started to initiate the reaction at 30 °C for 1 h, and identical solutions that were kept under dark served as blanks. The absorbance was read at 560 nm in the spectrophotometer against the blank. SOD activity is expressed in U mg−1 protein (U = change in 0.1 absorbance h−1 mg−1 protein).
2.5.2 Peroxidase (POX, EC 1.11.1.7)
POX was assayed by the method of Kumar and Khan [31]. Assay mixture of POX contained 2 ml of 0.1 M phosphate buffer (pH 6.8), 1 ml of 0.01 M pyrogallol, 1 ml of 0.005 M H2O2, and 0.5 ml of enzyme extract. The solution was incubated for 5 min at 25 °C, after which the reaction was terminated by adding 1 ml of 2.5 N H2SO4. The amount of purpurogallin formed was determined by measuring the absorbance at 420 nm against a blank prepared by adding the extract after the addition of 2.5 N H2SO4 at zero time. The activity was expressed in unit mg−1 protein. One unit is defined as the change in the absorbance by 0.1 min−1 mg−1 protein.
2.5.3 Catalase (CAT, 1.11.1.6)
The activity of CAT was measured according the method of Chandlee and Scandalios [32] with small modifications. The assay mixture contained 2.6 ml of 50 mM potassium phosphate buffer (pH 7.0), 0.4 ml of 15 mM H2O2 and 0.04 ml of enzyme extract. The decomposition of H2O2 was followed by the decline in absorbance at 240 nm. The enzyme activity was expressed in units mg−1 protein (U = 1 mM of H2O2 reduction min−1 mg−1 protein). The enzyme protein was estimated by the method of Bradford [11] for all the enzymes.
For all the enzymatic calculations, the proteins were determined by the method of Bradford [33], using bovine serum albumin (BSA, Sigma, USA) as the standard. Each treatment was analysed with at least seven replicates and a standard deviation (SD) was calculated; data are expressed in mean ± SD of seven replicates.
3 Results and discussion
3.1 Oxidative damage
The H2O2 content (Fig. 1a) increased with the salinity treatment in shoot and root, the highest content being 90 DAS in root. Oxidative damage to tissue lipid was estimated by the content of total TBARS. The plants under treatments of NaCl showed a trend towards an increase of the TBARS content (Fig. 1b). LPO has been associated with damages provoked by a variety of environmental stresses [1,2]. Polyunsaturated fatty acids (PUFA) are the main membrane lipid components susceptible to peroxidation and degradation [34]. The increase in LPO can be correlated with the accumulation of ions and active oxygen species (AOS) production under salt stress [35]. The level of LPO, in case, indicates the extent of salt tolerance as reported by Bor et al. [36] in sugar beet and wild beet under NaCl treatment.
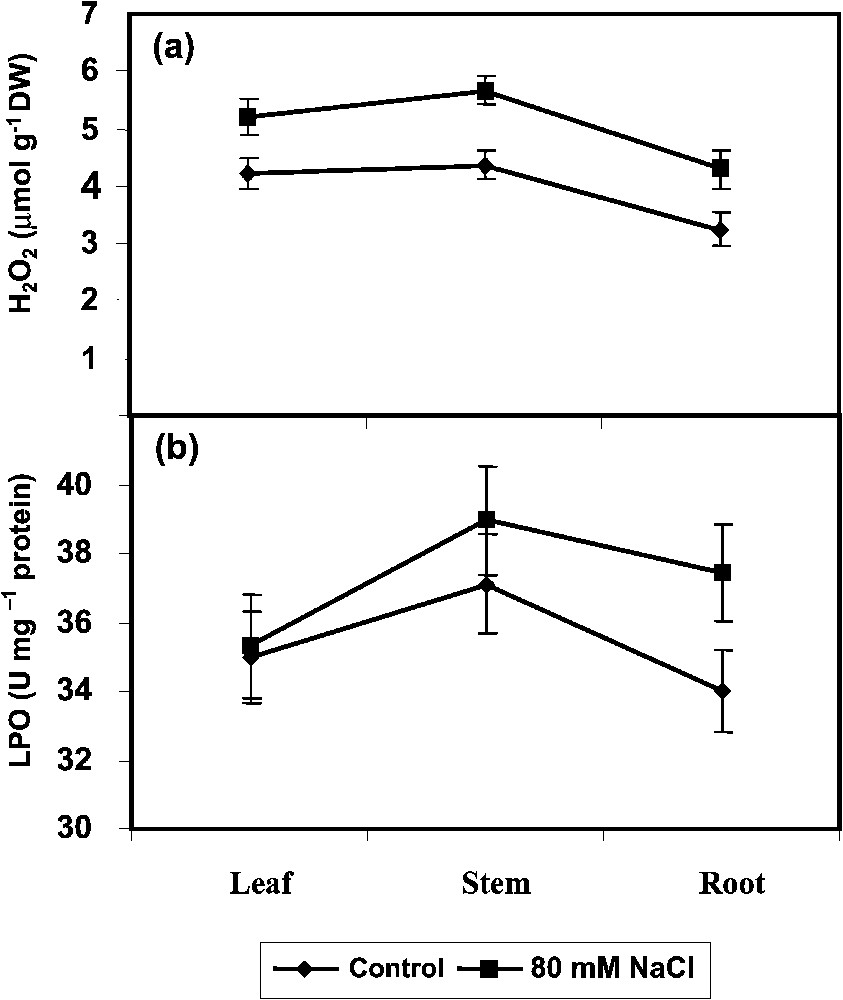
Effect of NaCl (80 mM) on (a) H2O2 accumulation and (b) lipid peroxidation (LPO) in Phyllanthus amarus on 90 DAS. Values are given as mean ± SD of seven replicates in each group.
3.2 Osmolyte concentration
In the present study, an increase in PRO accumulation in P. amarus under salinity, associated with a concomitant increase in γ-GK (PRO synthesizing enzyme) and a decrease in PROX (PRO degrading enzyme) activities (Fig. 2a) has been pointed out. One of the most important mechanisms exerted by higher plants under salt-stress conditions is the accumulation of compatible solutes such as GB. In the present study, the amount of GB increased with NaCl treatments in P. amarus plants (Fig. 2b). In majority of plants, salt stress leads to changes in gene expression, leading to an increased synthesis of osmoprotectors and osmoregulators [5]. Osmotic adjustment is the main component of the physiological machinery by which plants respond to soil salt stress. Free PRO and soluble sugar accumulation in the leaves under stress conditions is of utmost importance for plant adaptation during stress [1,7]. In most plants, there is an increased accumulation of amino acids and amines (e.g., PRO, B-alanine, GB) in their tissues in response to salt stress. The way these compounds are accumulated differs between species and ranges form only one to several different compounds being accumulated [37]. GB accumulation resulted from the NaCl-induced oxidative stress, and is helpful in the stimulation of salt-tolerance mechanisms [38]. The PRO metabolizing enzyme, γ-GK, increased under the NaCl salinity in P. amarus seedlings. This enzyme plays an important role in the synthesis of PRO. γ-GK activity can be inversely correlated with PROX activity and protein content in salt-treated plants. PRO accumulation in NaCl-stressed seedlings can be attributed in part to the increased level of γ-GK activity [39].
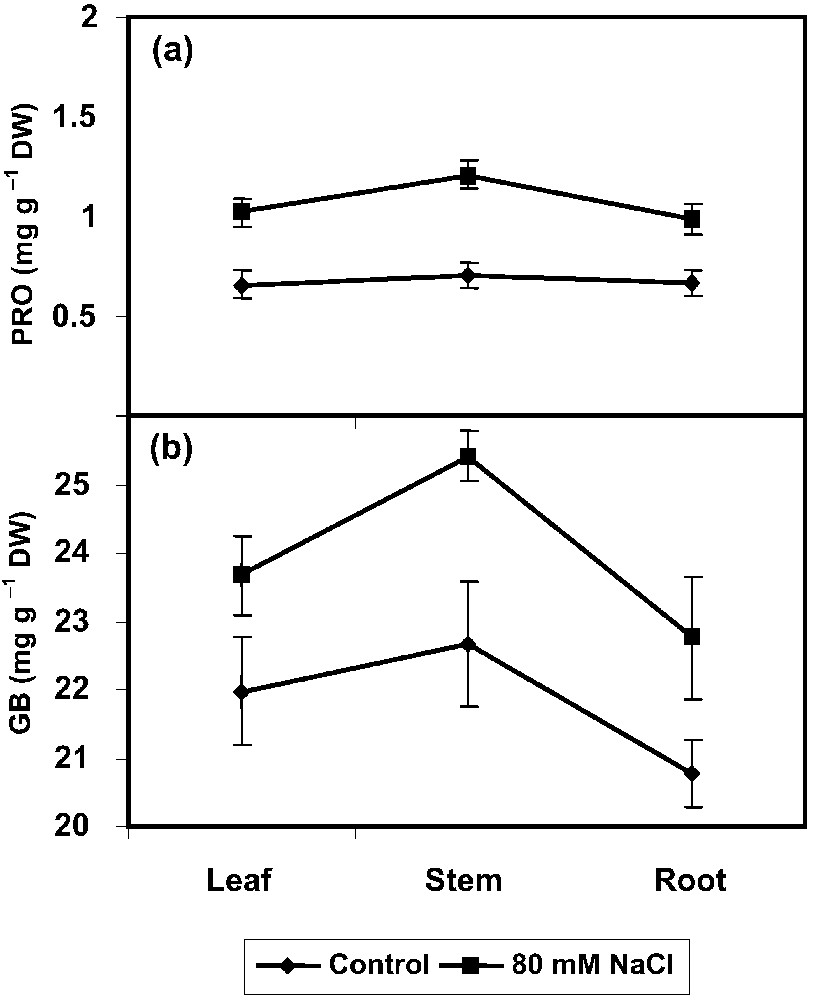
Effect of NaCl (80 mM) on (a) proline (PRO) and (b) gycinebetaine (GB) accumulations in Phyllanthus amarus on 90 DAS. Values are given as mean ± SD of seven replicates in each group.
3.3 Enzymes of proline metabolism
The γ-GK activity has been increased largely in shoot and root in the NaCl-stressed P. amarus plants when compared with control (Fig. 3a). NaCl has inhibited largely PROX activity in all parts of P. amarus when compared with control. PROX activity decreased under NaCl stress in P. amarus seedlings when compared to control (Fig. 3b). This enzyme converts free PRO into glutamate. Reduction in PROX activity and simultaneous increase in PRO level were reported in low-temperature-stressed wheat [40]. PROX oxidize the PRO and convert it back to glutamate. This enzyme also influences the level of free PRO. The activity of both PRO-degrading enzymes, PROX and proline dehydrogenase (PDH), were significantly inhibited in the salt-stressed green gram seedlings [41]. PRO may act as a non-toxic osmotic solute preferentially located in the cytoplasm or as an enzyme protectant, stabilizing the structure of macromolecules and organelles. Accumulated PRO may supply energy to increase salinity tolerance [1–3,7]. PRO, as an osmoprotectant compound, plays a major role in osomoregulation and osmotolerance [1]. However, its definite role in exerting salinity resistance continues to be a debate [38]. Although the precise role of PRO accumulation is still debated, PRO is often considered as a compatible solute involved in osmotic adjustment [42]. Anyway, understanding the biosynthesis, degradation, transport, and role of PRO during stress and the signalling events that regulate stress-induced accumulation is vital for developing stress-tolerant plants [43].
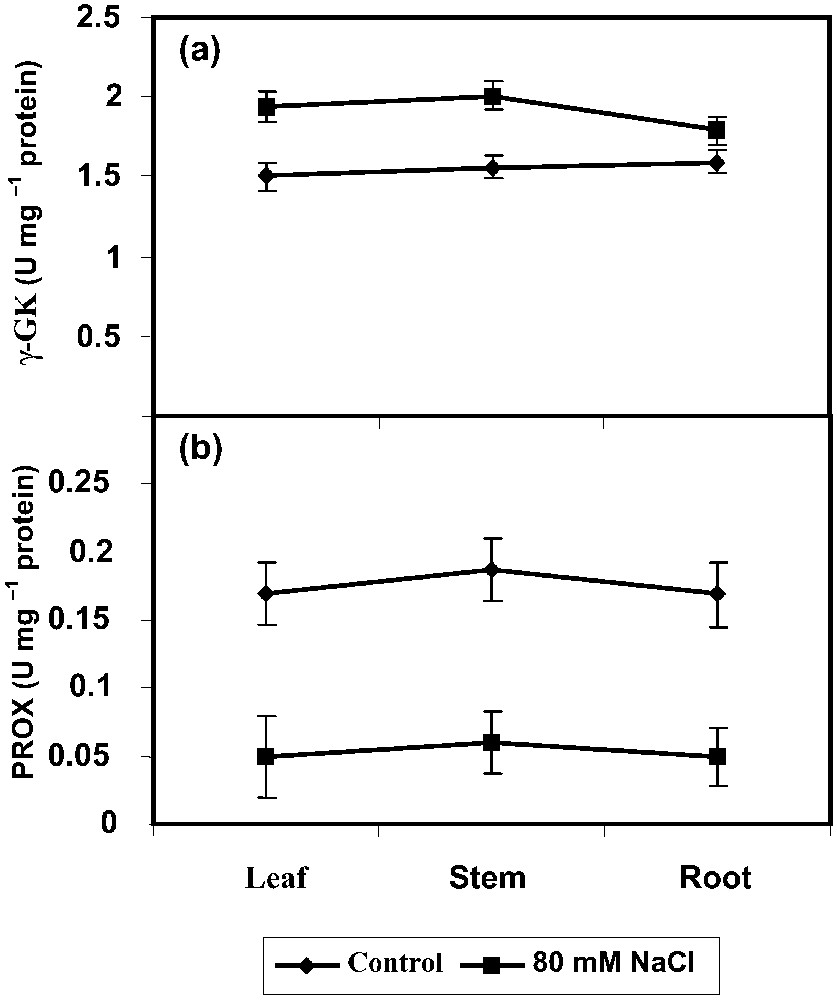
Effect of NaCl (80 mM) on (a) γ-glutamyl kinase (γ-GK) and (b) proline oxidase (PROX) activities in Phyllanthus amarus on 90 DAS. Values are given as mean ± SD of seven replicates in each group.
3.4 Activities of antioxidant enzymes
The SOD, POX, and CAT activities increased in all parts of the NaCl-stressed plants when compared to control (Fig. 4a–c). SOD activity directly modulates the amount of ROS. Similar results were seen in the case of CAT activity also. The unique importance of Ca2+ for stabilization of members is well known [44]. SOD and CAT activities have been reported to be negatively correlated with the degree of damage of plasmalemma, chloroplast, and mitochondrial membrane systems and positively related to the indices of stress resistance [45]. Increased antioxidant potential was reported previously in drought-stressed Vigna unguiculata plants under propiconazole treatment [19]. ROS scavenging depends on the detoxification mechanism provided by an integrated system of non-enzymatic reduced molecules, like AA and glutathione, and enzymatic antioxidants [9]. As part of this, antioxidant enzymes play important roles in the defence mechanism against oxidative stress. Antioxidant mechanisms may provide a strategy to enhance salt tolerance in plants, though the detailed mechanisms are not yet clear [10].
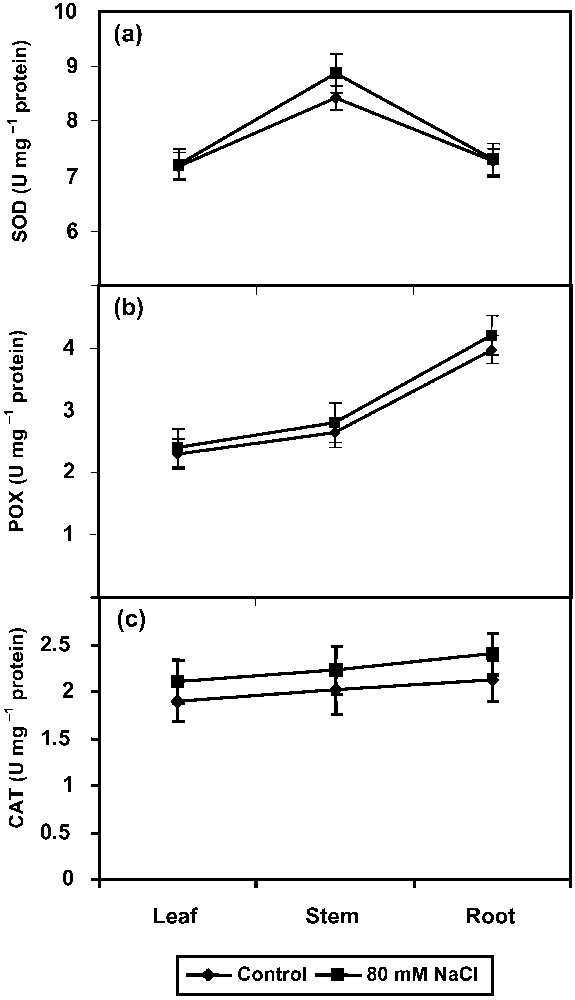
Effect of NaCl (80 mM) on (a) superoxide dismutase (SOD), (b) peroxidase (POX) and (c) catalase (CAT) activities in Phyllanthus amarus on 90 DAS. Values are given as mean ± SD of seven replicates in each group.
From these results, it is clear that plants under salt stress are highly regulated by components of both osmoregulation and antioxidative systems. Our results indicated that the cultivation of medicinal plants like P. amarus in saline areas would increase its PRO metabolism and defence mechanisms.