1 Introduction
Nature offers innumerable instances of symmetry, and because “symmetry is a theme that spans the human life world, technology, culture and nature” ([116]; see also [66,191]), the symmetry properties of organisms are particularly captivating for naturalists. Among others, the German biologist and polemicist E. Haeckel considered symmetry as one of the most fundamental properties of organism form, and as a primordial criterion for ranging the diversity of living beings into a hierarchy [87]. The recognised importance of symmetry in taxonomy was soon formalised in classifications separating radial and bilateral members of various groups. For example, at the beginning of the 19th Century, Cuvier [42] united all non-bilateral animals under the phylum Radiata, and later Hatschek [91] created the formal group Bilateria (which included nevertheless the atypical pentaradial echinoderms, removed from the Radiata by Leuckart [114] in 1848). Other instances of symmetry-based systems include the classification of many plant taxa (since the work of de Candolle [28]), and the traditional classifications of diatoms and sea urchins. More generally, the developmental origin, physiological and ecological significance, and evolution of organism symmetry have been and remain important themes for researchers.
In this article, I propose a broad perspective on the evolution of symmetry and polarity in multicellular animals (Metazoa). Geometry was central in the classical notion of “type” [174] and still lies at the heart of the “body plan” concept, i.e. the functional architecture characterising each phylum. The recognition of about thirty animal phyla in recent textbooks (e.g. [27]) reflects the dazzling anatomical and embryological disparity existing among extant metazoans. Although in recent years, our views on animal evolution have been deeply renewed by decisive progress in reconstructing the animal phylogenetic tree [64,89,111,185] and by comparative data on the genetic mechanisms underlying the construction of body plans (the evolutionary developmental biology or “evo-devo” approach) [9,30,144], many important evolutionary questions remain opened, particularly with respect to the evolution of body plan geometry. Compare, for example, a sea anemone and a fly. Despite their radically different constructions, you may seek for some correspondence between the geometrical elements of their bodies (e.g. are their main body axes homologous, i.e. derived from the main body axis of a remote common ancestor?). You may also ask if the radial symmetry of the sea anemone is ancestral with respect to the bilateral symmetry of the fly (in zoology textbooks, you would often find a positive answer).
Thus, after having reviewed the major types of symmetry existing among adult metazoans and how they relate to polarities, I will address the difficult problem of reconstructing transitions between types of symmetry during evolution, from the point of views of comparative anatomy, phylogeny, and evo-devo. I will also discuss the homology of polarity axes, in the light of recent developmental gene data. The taxonomic perspective in this paper is very broad and corresponds to the so-called “base of the metazoan tree” which in practice means that I will essentially compare the sponges, placozoans, ctenophores, cnidarians and bilaterians, while topics that specifically concern the Bilateria will not be developed (e.g. origin of pentaradial symmetry in echinoderms [85,142,143,195]; evolution of left-right dissymmetries in the context of bilaterality [58,153,154]). Although additional ancient animal lineages with interesting symmetry patterns occur in the Precambrian and early Paleozoic fossil record (see for example [65]), I prefer to leave them apart, not only because their analysis would require long developments, but also because their phylogenetic affinities with modern phyla are controversial, obscuring their significance with respect to the early evolution of animal symmetry.
2 Definition of symmetry and polarity
While in the late 19th Century and early 20th Century the formal description of organism geometry was developed by some authors to the extent that it was recognised as its own discipline (called “promorphology” by Haeckel [87]), this approach has totally disappeared in the modern literature (with rare exceptions, e.g. [15]), and geometry concepts applied to the animal form (such as types of symmetry) tend to receive only approximate (often incorrect) definitions in textbooks and articles. In parallel, there is a tendency among biologists to depreciate abstract morphology, as if it was nothing more than a “futile exercise” [137]. However, rigorous geometrical concepts are necessary for translating form into words [47] and thus for understanding the evolutionary and developmental issues that deal with them. The application of geometrical abstractions to the form of organisms requires some level of approximation, but contrary to occasional claims (e.g. [137]; see also discussion in [47], Ch. 17) this does not affect their relevance nor interest.
In common usage, symmetry denotes the balanced distribution of equivalent or corresponding entities. Symmetry is also a mathematical concept, of central importance in the algebraic group theory, and of very broad application [116]. The particular cases of rotational and reflection symmetries in the Euclidean space will be sufficient for our purpose, although a more general theory of organism geometry would require other kinds of symmetries (e.g. translational, helicoidal, ...) to be considered. Rotation of axis Δ and angle θ is a transformation which maps any point M to M′ with angle , where O is the orthogonal projection of M on the axis. Reflection maps any point to its mirror image with respect to a reference point, line or plane. For example, in the reflection of centre O, any point M is mapped to M′ such as O is the centre of the segment [MM′]. Symmetry of an object or a figure is invariance in one or several such transformations. For example, a sphere has reflection symmetry, because it is invariant in reflection with respect to its centre or to any line or plane that contains it, and it has rotational symmetry, since it is invariant in any rotation whose axis contains the centre. This does not mean that points of the figure are individually invariant, but that all of them map onto the figure.
The polarity concept as commonly used by biologists is more difficult to define in rigorous geometrical terms. Intuitively, a structure is said to be polarised if it is different at one extremity (or pole) with respect to the opposite one. A spoon, for example, is polarised, with a flat pole used to handle the spoon and an enlarged, concave pole to pour the soup. The concept, however, does not apply only for both extremities along the direction of main length. For example, the marked difference between both spoon surfaces (concave vs. convex) is also polarity. Thus, differentiation of morphology between both extremities of a segment that crosses the organism entirely is body (or general) polarity. In addition, internal polarities (along segments that do not cross the entire body) may occur at any spatial scale. Note that the same word “axis” is used to design two very different things: lines with the property of being symmetry axes (either of reflection and/or rotational symmetry) and polarity axes used to describe the spatial variation of morphology. It is important to understand that while the number of points, axes or planes of symmetry is strictly determined for any given figure, the number of polarity axes is theoretically unlimited, because an infinite number of reference segments are always a priori possible. However, the important thing is the minimal number of polarity axes required to follow the three-dimensional variations of morphology across the organism in the easiest way. A corollary is that the choice of polarity axes (not their minimal number) is more or less arbitrary, even if dictated to a large extent by the construction and biology of organisms. A further complication stems from the fact that anatomists recognise in some animals “modified” body polarity axes (by reference to related animals having “normal” axes) that are not straight but more or less curved. For example, cephalopods and gastropods are interpreted as modified molluscs with a U shaped antero-posterior axis, the posterior pole represented by the anus being close the mouth at the anterior pole. In these cases, there is discrepancy between anatomical axes and pure geometrical axes [137]. However, this mostly concerns derived bilaterians (additional examples include the bryozoans and sipunculans) and will not be developed further here.
3 Types of symmetry and body polarity axes: the promorphology perspective
Three major fundamental types of symmetry are classically recognised among adult metazoans (asymmetrical, radial, bilateral), to which adds the more anecdotical spherical symmetry [23,27,37,61,87,98,169]. I propose hereafter a revised classification of the types of symmetry for multicellular animals (Fig. 1) comprising five distinct types instead of four, because I deem necessary to separate strictly cylindrical (or axial) symmetry from radial symmetry, for reasons that I explain below. In the following, I do not intend to promote a typological view of animal symmetries, but only to formulate clear definitions of the character states, as a starting point for the subsequent discussion on their evolutionary patterns.
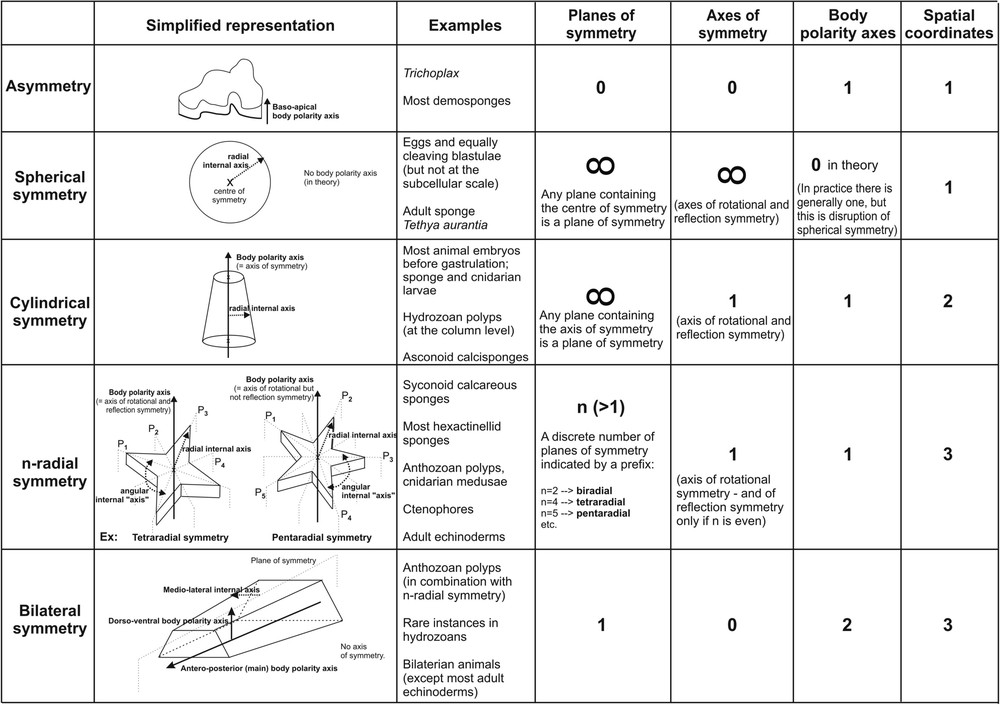
The five major types of symmetry recognised in this study for the Metazoa, with their defining geometrical properties and a few examples. Note that the number of coordinates (last column) is the sum of the number of body (or general) polarity axes and of the number of internal polarity axes.
Les cinq types de symétrie principaux reconnus dans cette étude pour les métazoaires, avec indication des propriétés géométriques qui les définissent et de quelques exemples. Remarque: le nombre de coordonnées (dernière colonne) correspond à la somme du nombre d'axes de polarité corporelle (= générale) et du nombre d'axes de polarité interne.
Organisms that lack any point, axis or plane of symmetry are said asymmetrical (Fig. 1). Examples include the majority of the sponges belonging to the class Demospongiae (Fig. 2A and B), and the simple amoeboid Trichoplax (“phylum Placozoa”) (Fig. 2D), in addition to a few extremely derived parasitic forms (e.g. the plasmodium of the Orthonectida). However, in encrusting demosponges (Fig. 2A) as in Trichoplax (Fig. 2D) the body shows clear morphological differentiation between the basal side attached to the substrate and the upper side, as reflected by histology (e.g. differing characteristics between the upper and lower epithelia) and by strong baso-apical differentiation of the skeleton architecture in many encrusting demosponges (e.g. Fig. 2C). Thus, these organisms have a (single) body polarity axis, called the baso-apical axis (Fig. 1), and their asymmetry does not imply absence of a structural plan. In addition, demosponges have internal symmetries around the oscula (see Fig. 2A) and non-encrusting demosponges (e.g. Fig. 2B) have more or less complex, species-specific general shapes, and their internal anatomy (notably with respect to skeleton architecture) is more or less sophisticated, with a variable number of internal polarity axes. Regarding terminology, “asymmetry” is a potentially misleading term because it might have two totally different meanings depending on the context. In this article, I use “asymmetry” in the strict sense (i.e. “asymmetry of indefinition”, [15]), but this word is also frequently used for secondarily disrupted symmetry (e.g. left-right disruption of bilaterality in vertebrates or other Bilateria [58,153,154]), although in this latter case “dissymmetry” is certainly more appropriate [15] (note that the same word, sometimes spelled “disymmetry”, has also been employed as a synonym of biradiality [8,98] but this use should be dismissed).

Examples of asymmetrical metazoans. A: Hexadella racovitzai (Demospongiae). B: Aplysina cavernicola (Demospongiae). C: vertical section in the body of the encrusting sponge Protosuberites prototipus (Demospongiae) showing clear baso-apical differentiation of the skeleton architecture (cho: choanosomal skeleton; ect: ectosomal skeleton; sub: substrate). D: Trichoplax adhaerens (Placozoa) (the blue staining reveals Not expression) (A and B: pictures taken during the Porifera Training Course in Marseille, July 2005, respectively by Bernard Picton and Thierry Perez; C: adapted from [181]; D: from [126]).
Exemples de métazoaires asymétriques. A : Hexadella racovitzai (Demospongiae). B : Aplysina cavernicola (Demospongiae). C : coupe verticale dans le corps de l'éponge Protosuberites prototipus (Demospongiae) montrant une nette différenciation baso-apicale de l'architecture du squelette (cho : squelette choanosomal ; ect : squelette ectosomal ; sub : substrat). D : Trichoplax adhaerens (Placozoa) (le marquage bleu correspond à l'expression du gène Not) (A et B : photographies réalisées pendant le « Porifera Training Course » à Marseille en juillet 2005, respectivement par Bernard Picton et Thierry Perez ; C : adapté de [181] ; D : extrait de [126]).
Spherically (or centrally) symmetrical organisms have one point of symmetry and an infinite or multiple number of axes and planes of symmetry (Fig. 1). Whereas spherical symmetry is rather common among unicellular eukaryotes (and a few multicellular ones, e.g. the green alga Volvox) [15], it is almost inexistant among adult metazoans, apart from exceptional instances of centrally radiating skeleton architecture in demosponges (e.g. genus Tethya, Fig. 3). Even in this case, spherical symmetry is not perfect, but is disrupted by a general baso-apical polarity axis, the sponge being attached to a substrate.

Example of a spherically symmetrical sponge, Tethya aurantium (Demospongiae). On the right, intact sponge (alcohol preserved); on the left, the sponges has been partially dissected to show the skeleton tracts (made of spongin and siliceous spicules) radiating from the centre. Note in addition that the sponge body is covered by a cortex containing a special skeleton (scale bar: 1 cm).
Exemple d'une éponge à symétrie sphérique, Tethya aurantium (Demospongiae). A droite, éponge intacte (fixée en alcool) ; à gauche, l'éponge a été partiellement disséquée pour mettre en évidence les fibres (constituées de spongine et de spicules siliceux) qui rayonnent depuis le centre. Remarquer par ailleurs que le corps de l'éponge est recouvert d'un cortex, renfermant un squelette particulier (barre d'échelle : 1 cm).
In cylindrical (= axial) symmetry, there is a single symmetry axis, invariance in all rotations that contain this axis, and an infinite number of planes of reflection symmetry (all planes that contain the axis of symmetry). Cylindrical symmetry is rare among adult metazoans, the only clear instances being calcisponges with an asconoid aquiferous system (Fig. 4A) and the body column of cnidarian polyps in the classes Hydrozoa (Fig. 4B) and Cubozoa. More arguably, the Trichoplax body (Fig. 2D), which I have presented above as asymmetrical (due to the lack of a defined shape), may also be viewed as cylindrically symmetrical, if an “ideal” circular body outline is postulated, independent from body contractions and movements.

Examples of cylindrically symmetrical metazoans. A: transverse section in Leucosolenia variabilis (Calcispongia), an asconoid calcareous sponge (atr: atrial cavity; cho: choanoderm; sp: spicules). The thin external epithelium or pinacoderm is not visible (scale bar: 100 μm). B: transverse section in the body column of Hydra (Hydrozoa, Cnidaria) (ect: ectoderm; end: endoderm; ga: gastral cavity; mes: mesoglea; nem: nematocysts) (scale bar: 50 μm). C: longitudinal section in the planula larva of Chrysaora (Scyphozoa, Cnidaria) (ect: ectoderm; end: endoderm). D: longitudinal section in the early gastrula of Serpula (Annelida, Bilateria) (al: archenteron; an: anus) (A and B: original pictures; C and D: from [10]).
Exemples de métazoaires à symétrie cylindrique. A : coupe transversale de Leucosolenia variabilis (Calcispongia), une éponge calcaire asconoïde (atr : cavité atriale ; cho : choanoderme ; sp : spicules). Le fin épithelium externe ou pinacoderme n'est pas visible (barre d'échelle : 100 μm). B : coupe transversale dans la colonne d'Hydra (Hydrozoa, Cnidaria) (ect : ectoderme ; end : endoderme ; ga : cavité gastrique ; mes : mésoglée ; nem : nématocystes) (barre d'échelle : 50 μm). C : coupe longitudinale dans la larve planula de Chrysaora (Scyphozoa, Cnidaria) (ect : ectoderme ; end : endoderme). D : coupe longitudinale dans une jeune gastrula de Serpula (Annelida, Bilateria) (al : archentéron ; an : anus) (A et B : images originales ; C et D : extrait de [10]).
n-radial symmetry corresponds to the repetition (n times) around an axis of the same set of anatomical structures (called an antimere), which in mathematical terms corresponds to invariance in n rotations. There is a single axis of symmetry and a discrete (n) number of planes of symmetry (Fig. 1). The n value is usually indicated by a prefix. For example, tetraradially symmetrical bodies (e.g. most cnidarian medusae) admit four symmetry planes and are invariant in four rotations. Radially symmetrical animals include many hexactinellid sponges (Fig. 5A), calcisponges with a syconoid aquiferous system (Fig. 5B), homoscleromorph sponges (Fig. 5C and D), ctenophores (Fig. 5E), most cnidarians (Fig. 5F), and, among the Bilateria, adult echinoderms. A curious geometrical property of radial symmetry is that the axis of rotational symmetry is an axis of reflection symmetry if n is even (for example, medusae are tetraradial and thus admit an axis of reflection symmetry), but not if n is odd (see Fig. 1: the mirror images of the sea star arms with respect to the rotational axis are located out from the body). However, this observation is certainly of limited relevance in biological and developmental terms.

Examples of radially symmetrical metazoans. A: external morphology of the siliceous skeleton of the glass sponge Euplectella (Hexactinellida). Note that in addition to multiradial symmetry, this complex skeleton architecture also shows translational and helicoidal symmetries. B: transverse section in Sycon raphanus (Calcispongia), a syconoid calcareous sponge (atr: atrial cavity; cc: choanocyte chamber; dc: distal cone) C: transverse section in an asexual reproductive bud of Oscarella tuberculata (Homoscleromorpha) (atr: atrial cavity; cc: choanocyte chamber), showing a syconoid-like organisation of the aquiferous system. D: drawing of the adult body of Oscarella tuberculata in section (Homoscleromorpha), showing the sylleibid organisation of the aquiferous system, i.e. with spherical choanocyte chambers branched to radiating exhalant canals. The detailed picture (box) corresponds to the section plane indicated by the double arrow on the drawing (cc: choanocyte chamber; emb: embryo; exc: exhalant cavity; exh: exhalant canal; inh: inhalant canal). E: Pleurobrachia pileus (Ctenophora), aboral view showing elements of octoradial symmetry (the eight comb rows, indicated by stars) and biradial symmetry (gt: tentacle sheath; ten: tentacle; and the polar fields indicated by the arrowheads). Elements of tetraradial symmetry are not visible. The arrow points to the apical sense organ. F: young medusa (post-ephyra) of Aurelia aurita (Scyphozoa, Cnidaria) (ga: gastrovascular canal; la: lappet; ma: manubrium; rho: rhopalium, a sensory organ; ten: tentacle). The symmetry here is octoradial but the internal anatomy at the level of the manubrium is tetraradial (not visible). (A, B, E, F: original pictures; C and the boxed picture in D: courtesy of Alexander Ereskovsky; D from [176].)
Exemples de métazoaires à symétrie radiaire. A : morphologie externe du squelette siliceux de l'éponge de verre Euplectella (Hexactinellida). Remarquer qu'en plus de la symétrie multiradiaire, l'architecture complexe de ce squelette est dotée de symétrie translationelle et de symétrie hélicoïdale. B : coupe transversale dans Sycon raphanus (Calcispongia), une éponge calcaire syconoïde (atr : cavité atriale ; cc : chambre choanocytaire ; dc : cône distal). C : coupe transversale dans un bourgeon de reproduction asexuée d'Oscarella tuberculata (Homoscleromorpha) (atr : cavité atriale ; cc : chambre choanocytaire), montrant une organisation du système aquifère de type syconoïde. D : schéma d'une coupe dans le corps d'Oscarella tuberculata (Homoscleromorpha), mettant en evidence l'organisation sylléïbide du système aquifère (chambres choanocytaires sphériques associées à des canaux exhalant à disposition radiaire). L'image de détail (encadré) correspond au plan de coupe indiqué par la double flèche sur le dessin (cc : chambre choanocytaire ; emb : embryon ; exc : cavité exhalante ; exh : canal exhalant ; inh : canal inhalant). E : Pleurobrachia pileus (Ctenophora), vue aborale montrant des éléments de symétrie octoradiaire (rangées de peignes, indiquées par les étoiles) et de symétrie biradiaire (gt : gaines tentaculaires ; ten : tentacules ; ainsi que les champs polaires indiqués par les têtes de flèche). Les éléments de symétrie tétraradiaire ne sont pas visibles. La flèche pointe sur l'organe sensoriel apical. F : jeune méduse (post-éphyrule) d'Aurelia aurita (Scyphozoa, Cnidaria) (ga : canal gastrovasculaire ; la : bractée ; ma : manubrium ; rho : rhopalie, un organe sensoriel ; ten : tentacule). Ici la symétrie est octoradiaire, mais en anatomie interne au niveau du manubrium elle est tétraradiaire (non visible sur l'image). (A, B, E, F : images originales ; C et l'image encadrée en D : images originales fournies par Alexander Ereskovsky ; dessin en D de [176].)
The distinction between cylindrical and radial symmetries is ignored in the zoology literature, both being confounded under the term “radial symmetry” (with cylindrical symmetry sometimes qualified as “full” or “perfect” radiality [27,193]). The regrettable consequence of this confusion is to conceal the specific problem represented by the repetition of parts (multimery) around an axis, a situation yet recognised by many 19th Century writers [13,62,87] as analogous to the repetition of parts along an axis (i.e. metamery or translational symmetry) [174]. Cylindrically symmetrical animals have no such repetition of parts. In addition, radial symmetry is not necessarily just cylindrical symmetry with something more, as exemplified by the echinoderms, which clearly became pentaradial starting from bilaterality, not cylindrical symmetry. The restricted use of the term “radiality” which I propose here has historical justification in the classical concept of the “radius”, the plane of symmetry of the repeated unit or antimere in radially symmetrical animals [15]. Now, although they are distinct types, cylindrical and radial symmetries have important axial properties in common. In both types there is a single axis of symmetry (= monaxonic organisation) which in all concerned metazoans is also the single (oral–aboral or baso-apical) body polarity axis. The developmental and evolutionary issues associated with this monaxonic heteropolar organisation are clearly distinct from that of the repetition of parts.
Bilateral symmetry is simply defined by the existence of a single symmetry plane (Fig. 1), which divides the body into two mirror halves. There is no symmetry axis. As a consequence, there are two orthogonal body polarity axes. Bilateral symmetry is particularly pronounced in the Bilateria, where the body polarity axes are called antero-posterior (AP) and dorso-ventral (DV). Besides the Bilateria, bilaterality also occurs in some cnidarians (see later).
Bilateral symmetry has often been mistakenly confounded with biradial symmetry [41,83,125,155,193] or at least bilaterality and biradiality have been put side by side (e.g. [98,124,173] and many others), which is not justified in my opinion. First, biradiality need not be considered as a distinct type of symmetry (contra Hyman [98] and most subsequent textbooks) since it is just n-radial symmetry with . More importantly, it has absolutely nothing to do with bilaterality. Biradial animals (e.g. ctenophores) have a single body polarity axis, which in biological and developmental terms represents the fundamental difference with bilaterality (two body polarity axes). Moreover, biradial animals are truly multimeric, with one or several type(s) of strictly identical (i.e. superimposable) twice-repeated structures around the axis (see Fig. 5E), whereas in a bilateral animal, the two mirror-image halves are not superimposable and thereby are recognisable as respectively the right and left half. Instances of dorsal-ventral symmetry evoking biradiality in some bilaterian phyla (e.g. nematodes) are probably derived rather than ancestral among the Bilateria and cannot be taken as an indication that bilaterian ancestors ever passed through a biradial state. Hence, I reject the idea that biradiality would be “intermediate” between n-radial () and bilateral symmetry [124,125], except possibly in the very particular case of the pharynx of anthozoan polyps (see later).
I have described the various symmetry types independently, but in fact they frequently occur in combination within the same organism, since different subsets of morphological structures might follow different types of symmetry. For example, different systems of radial symmetry frequently coexist, generally in concordance (e.g. octo-, tetra- and biradial symmetry in ctenophores, Fig. 5E). There are also instances of co-occurrence between cylindrical and radial symmetry (e.g. cylindrical body column vs. radially disposed peri-oral tentacles in hydrozoan polyps), and between radial and bilateral symmetry. An example of the latter is offered by the sea stars, in which the global pentaradial symmetry is accompanied by the differentiation of one out of the five genital plates into the madreporite plate (where the ambulacral system communicates with the exterior), an element of bilateral symmetry. Among echinoderms, combination of bilateral and pentaradial symmetry is even considerably more pronounced in sea cucumbers and in irregular sea urshins. Likewise, as I will explain in more details later, most anthozoans combine radial and bilateral symmetry.
In the preceding overview of the symmetry types of metazoan body plans, I have only considered basic adult organisation, leaving apart the symmetry of early ontogenetic stages. Describing the symmetry types of embryos faces specific difficulties since their geometry might be grasped at several different scales, depending on whether intracellular differentiations are taken into account or not, and on whether cells are considered as geometrical objects, or are mentally fused with each other into an abstract embryonic form. Cleavage patterns have strong evolutionary significance, but will not be dealt with here because they have little direct connection with the global symmetry of the adult body plan. Among the symmetry types defined above for adult metazoans, spherical symmetry is more or less recognisable in eggs and some early animal embryos (equally cleaving blastulae), although this requires a high level of abstraction to neglect not only subcellular polarities, but also the detailed spatial distribution of individual cells throughout cleavage stages. In addition, many animal embryos cleave unequally and therefore are absolutely not spherical once the egg has started to divide. In contrast, all animals pass through a clear cylindrical global morphology at some time during their ontogeny, as illustrated for example by sponge and cnidarian post-gastrulation embryos and larvae (Fig. 4C), ctenophore embryos before organogenesis, and most embryos before gastrulation in the Bilateria (Fig. 4D).
4 On the biological significance of the various types of symmetry
It has been repeatedly stated that each of the symmetry types is connected with a definite type of environment and with a definite type of lifestyle (e.g. most zoology textbook associate radial symmetry with fixed benthic life style and bilateral symmetry with free-swimming). I argue here that empirical evidence contradicts this idea and demonstrates instead that there is no simple rule linking each type of symmetry to a particular life style or environment.
Thus, cylindrical symmetry has totally different adaptive values in non-bilaterian larvae (where it is associated with directional locomotion without preferential orientation with respect to gravity), in asconoid calcisponges (where it corresponds to unidirectional water flow within a simple fixed tube) and in the body column of hydrozoan and cubozoan polyps (where it is the mere consequence of diploblasty, small size and anatomical simplicity).
Radial symmetry is a form of multimery (repetition of parts) in which all parts are faced with equal environmental characteristics. Apart from that, radiality has totally disparate adaptive values among concerned groups. The radial symmetry of syconoid sponges (Fig. 5B–D) results from a particular mode of aquiferous system organisation, and thereby its functional significance relates to hydrodynamics [118]. The complex multiradial architecture of the hexactinellid sponge skeleton (Fig. 5A) is designed to provide mechanical stability at minimal cost [5]. The radial arrangement of tentacles in cnidarian benthic polyps and pelagic medusae allows prey capture in all directions. There are also instances of radially disposed structures used in locomotion, either unidirectional (as for the ctenophore comb rows) or multidirectional (as for the sea star arms). In still other cases, radial external ciliated structures are used for generating vertical water currents. This is the main functional significance connected with the multiple convergent acquisitions of more or less pronounced radial anatomies in the Bilateria (e.g. lophophore in bryozoans, arms in sabellid polychaetes or in crinoid echinoderms).
Bilaterality is usually said to be fundamentally linked to directional locomotion with preferential orientation with respect to gravity, but in fact this is true only of the Bilateria, with the AP axis generally corresponding to the direction of main body length and to the direction of locomotion (with the mouth located anteriorly), while the DV axis is usually oriented with respect to gravity, the ventral side facing the substrate. This particular significance of bilaterality in the Bilateria may in part explain why they are the only animals present in terrestrial environments, where gravity has a much stronger impact than in water. There are, however, many instances of derived bilaterians having lost directional locomotion while retaining bilaterality (e.g. mussels, barnacles, tapeworms, ...), suggesting that in these cases the bilateral symmetry might have alternative aptive values.
In addition to bilaterians, many cnidarians (among Anthozoa and Hydrozoa) also have bilateral anatomies, which interestingly have various functional significances depending on the taxa, but are never linked to directional locomotion. Thus, bilaterality in cnidarians may be connected for example with polyp fixation on a vertical support, combined with preferential top-down orientation, as in the case of many solitary members of the fossil anthozoan taxon Rugosa [152] and of zoids in some hydrozoan colonies showing differentiation with respect to the axis of the colony (e.g. swimming bells of siphonophores [29]; gastrozoids of sertulariid thecates [21]); with fixation along the margin of the tube of a commensal polychaete, with localisation of the tentacle insertion points on the side facing the centre of the tube, in the two-tentacle hydrozoan polyp Proboscidactyla [21]; with the restriction of tentacle production in a localised growth zone (in the giant hydrozoan Branchiocerianthus); with the possession of a single tentacle in some hydrozoan medusae (for a detailed illustrated account on these occasional instances of bilaterality in the Hydrozoa, see Beklemishev [15]); or with the creation of diametrally opposed internal water currents in many anthozoan polyps (see later).
That each of the symmetry types may be associated with various ad hoc functionalities suggests that symmetry is not strictly constrained biologically, and that body symmetry was affected by multiple adaptive and/or exaptive changes during evolution.
5 Did evolutionary transitions between symmetry types reflect shifts in organism complexity? A morphological, developmental and genomic perspective
Throughout the modern zoological and evo-devo literature it is more or less explicitely assumed that during early animal evolution, adult symmetry evolved from asymmetrical to radial (cylindrical symmetry being ignored), and then to bilateral [6,27,70–72,94,97,98,108,124,169,173]. These would represent three essentially distinct levels of organisation that were successively reached in a stepwise fashion, each step corresponding to an increase in body complexity, and as such representing an outstandingly important event in the course of animal evolution. I will examine later on in the following section how comparative anatomy in a phylogenetic framework allows re-evaluating this “conventional scenario”. In the present section, I intend to compare the various types of symmetry in terms of “complexity”, from the morphological point of view and then from the developmental point of view, in order to understand the potential significance of transitions from one type of symmetry to the other. Two levels of interrogation are identified. Firstly, does transition from a symmetry type to another imply per se a shift in morphological complexity? In case of a positive answer, the second pivotal interrogation is whether or not any transition to a more complex type necessarily reflects a shift with respect to intrinsic organism properties, i.e. genetic complexification, and/or the elaboration of more complex developmental programs (as implicitely assumed in the conventional scenario).
5.1 The promorphological complexity of symmetry types
There was a long tradition for ranging types of symmetry in graded series thought to reflect increasing complexity. High degrees of symmetry (i.e. high numbers of symmetry planes or axes) were regarded as reflecting primitiveness, because “the most highly symmetrical systems are also the most random” [137], as illustrated in the non-living world by self-organising systems such as crystals (see [11] for physical aspects and [174] for the historical influence of crystallography on 19th Century biologists and their considerations about symmetry). Evolution was expected to increase heterogeneity, thus decreasing the number of identical parts. This is well exemplified by Beklemishev's views, [15], on the evolution of protozoans (but according to this author the same principles apply to metazoans). Above protozoans showing asymmetry of indefinition (e.g. amoebae), the most primitive grade was represented by perfectly spherical taxa, evolving towards reduction of the number of symmetry axes while keeping a general spherical shape. The following grade comprised cylindrical organisms, evolving from non-polarised (“homopolar”) to bipolarised (“heteropolar”) and from a large number of planes of symmetry towards a reduction of this number. Bilateral (or even dissymetric) protozoans were considered as the most evolved forms. The conventional scenario for metazoans outlined previously has clear roots in the same philosophy, even if the series is shorter because symmetry types are much less diverse in multicellular animals than in unicellular eukaryotes.
An alternative approach to the contribution of symmetry to morphological complexity is to consider for each of the symmetry types the minimal number of degrees of freedom that constrain the variation of morphology in the three-dimensional space. Here we need to take into consideration not only the body (or general) polarity axes, but also those internal polarity axes that are inherent to each symmetry type (dashed lines in Fig. 1). The sum of internal and body polarity axes (last column in Fig. 1) constitute a full system of coordinates, meaning that morphology at any given point within the organism is unambiguously determined, once these coordinates are fixed. In flat asymmetrical animals, as in spherically symmetrical ones, morphology is determined by a single coordinate (distance with respect to the substrate/to the centre). In cylindrical symmetry, morphology varies along the oral–aboral polarity axis and along the radial internal axis (e.g. succession, from the axis to the periphery of hydrozoan polyps, of the gastral cavity, endoderm, mesoglea and ectoderm, Fig. 4B). In radial symmetry, the same two coordinates are required, but in addition morphology varies in an angular fashion. Thus, there is a third (angular) coordinate (Figs. 1, 5). Although this is not an “axis” in the proper sense, it might be called the internal “angular axis” by analogy. To describe a bilaterally symmetrical animal, three systems of spatial coordinates are needed: position along the AP and DV body polarity axes, and position along the medio-lateral internal axis (Fig. 1) (left-right disruption of the bilaterality corresponds to the addition of a fourth coordinate).
Thus, according to the number of spatial directions in which morphology varies, symmetry types can be classified as “monodirectional” (asymmetrical and spherical), “bidirectional” (cylindrical) and “tridirectional” (radial and bilateral), and this strictly reflects the contribution of symmetry to morphological complexity. In addition, this way of characterising symmetry type complexity is directly connected to developmental issues (i.e. how morphological differentiation between cells that are located in different areas is generated during development). Putting aside the case of asymmetry, there is a clear albeit imperfect inverse correlation between the number of symmetry elements (axes or planes) and the number of polarity axes (general or general + internal) (compare the last four columns in Fig. 1), in consistence with the common statement that polarity is breaking of symmetry. Interestingly enough, cylindrical and radial symmetries differ in number of coordinates, implying that they indeed correspond to two different levels of morphological organisation. In addition (and maybe counter-intuitively), bilateral symmetry does not appear intrinsically more complex than radial symmetry.
5.2 Symmetry types and developmental complexity
These considerations naturally lead us to ask if more complex types of symmetry necessarily require more complex developmental mechanisms for their establishment during ontogeny. Unfortunately, comparisons of developmental mechanisms across symmetry types are hampered by a critical lack of empirical knowledge, particularly concerning radial symmetry. Nevertheless, a few interesting ideas are emerging, based on our current understanding of the general features of polarity-generating mechanisms in animal development, and on recent advances in mathematical modelling.
In metazoans, the global symmetry of the body plan arises early during ontogeny within a relatively short time frame [37], contrasting with symmetrical patterns emerging through the progressive addition of similar body parts, under some specific spatial constraints, in some other multicellular eukaryotes (e.g. Fibonacci helicoidal symmetries in various vegetal structures [4,47,117]). Exceptions to this rule mostly concern geometrical patterns in animal colonies [16,63] and will not be discussed here. Animal body plan symmetry is the final outcome of a (rapidly progressing) succession of polarising (symmetry breaking) events occurring in the embryo, within single cells or within nested fields of cells. Thus, body plan symmetry by itself has no meaning in terms of developmental cellular/molecular processes, but is a by-product of a robust and invariant series of polarising mechanisms, among which the earliest defines the major body axes only because they are the earliest.
The molecular mechanisms underlying the establishment of polarity within a cell field are remarkably similar throughout the Metazoa. Leaving apart the probably derived instances of highly mosaic developments (where early cell fate choices and thus axis specification requires little cell-cell interactions; e.g. in ctenophores [123] or in Drosophila [80]), the fundamental polarity-generating mechanism involves an inductive signal mediated by one or several (often antagonising) diffusing molecules (morphogenes), which through a signalling pathway provide instructive clues for genes to be expressed differently in cells located in different spatial areas. There are a limited number of major signalling pathways, among which, for example, the well-studied Wnt, TGF-β, Hedgehog (Hh) and Notch/Delta pathways (designated by using the names of their ligands) [80]. During development, these signalling pathways are not only acting in the generation of early embryonic polarities, but are pleiotropically used and re-used in a large number of contexts and thus may determine polarities at every spatial scales and at any life stage.
Mathematical modelling have demonstrated that there is no simple relationship between the number of involved signalling molecules and the complexity of the resulting geometrical pattern. For example, in reaction–diffusion coupling models, more or less complex patterns are formed in epithelia through the dynamic interaction of only two diffusible factors, an activator and an inhibitor that is induced by the latter and diffuses faster [134,187]. Cummings [39–41] proposed a mathematical model coupling the dynamic distribution of two antagonising morphogenes and their receptors with shape changes in epithelial cell sheets. Under some parameters, he obtained a clear radially symmetrical pattern on a three-dimensional figure topologically equivalent to a gastrula (he called this figure a “Proto-Cnidaria”) (Fig. 7 in [41]). Astonishingly, what I described previously as a tridirectional type of symmetry can be developmentally reached through epithelial morphogenesis involving a single couple of antagonising diffusing molecules. This result has far-reaching implications, because exactly the same molecular device can under other constraints and parameters lead to monodirectional or bidirectional geometrical patterns, thus totally uncoupling morphological complexity from the complexity of underlying molecular developmental tools. Likewise, Cummings [41] claimed to have obtained bilateral symmetry (“Proto-protostome”) under still other parameters with the same model, but unfortunately what he called a bilateral pattern was in fact biradial (his Fig. 8), thus leaving unanswered whether or not bilaterality requires in theory more “developmental complexity” than radial symmetry does. Although at first sight it could seem evident that more signalling pathways are needed to create an additional polarity axis, this is not necessarily true since a single patterning system can work sequentially along separate axes (e.g. in vertebrates, Wnt signalling acts prior to gastrulation to define the dorsal organiser, and during gastrulation to posteriorise along the developing AP axis [133,134]).
5.3 Insights from genomes and conclusions
Recent comparative genomic analyses have revealed that virtually all families of signalling ligands, receptors and transductors were already established in the common metazoan ancestor. Cnidarian genomes have a high level of genetic complexity and contain most of the molecular developmental toolkit that bilaterians use to build their body plans [135,136,163,184]. Not only are the major gene families present, but the diversity of their members is often of the same order of magnitude than in bilaterian genomes (e.g. within the Wnt family [109]). Likewise, components of the Wnt, TGF-β, EGF, IGF, RTK, Notch/Delta, Hh and Jak/Stat signalling pathways have been identified in sponges [1–3,149]. Thus, there is no relationship between genetic and morphological complexity [12,109,184], and in particular, the common ancestor of Metazoa “already” possessed many more molecular signalling systems than required to achieve even the most “complex” types of symmetry.
The inescapable conclusion is that at no stage during animal evolution were transitions from one type of symmetry to another limited by molecular possibilities. Clearly, such transitions (regardless of their direction) did not involve genetic innovation, but simply a different deployment of existing molecules during ontogeny. Based on these considerations, I suggest that the frequency of changes in symmetry types during animal evolution was mostly dictated by physical factors [148] (the types of symmetry representing various solutions in the “game of possible” of animal geometry), structural constraints (the earliest developmental events that set up the body plan being more or less “locked in” by the dependence of later patterning events upon them) and by natural selection exploring the wide range of adaptive possibilities offered by the various types of symmetry, under different environmental circumstances or life styles.
6 Reconstructing the early evolution of symmetry types: a comparative anatomy perspective
The “conventional scenario”, postulating two major transitions during early animal evolution from asymmetry (represented by the sponges and Trichoplax) to radial symmetry (represented by the “coelenterate phyla”, ctenophores and cnidarians) and then to bilaterality (the Bilateria) [6,27,70–72,94,97,98,108,124,169,173], was inherited from the “traditional textbook phylogeny” (TTP) [101]. This long-standing theory of animal evolution postulated a graded increase in complexity from the earliest metazoans to present day “higher” animals. Recent molecular phylogenies of the Bilateria have refuted this simplist view and indicated that patterns of body plan character evolution are in fact much more complicated than expected under the TTP [89]. Notwithstanding, the “conventional scenario” of symmetry type evolution has remained almost invariably accepted, still in recent years, with only rare exceptions (e.g. [193]), and even the recent proposal, from developmental gene studies, that bilaterality would have predated the cnidarian/bilaterian divergence [72,75,127] does not represent more than a variation on the same paradigm.
6.1 The metazoan ancestor was probably not asymmetrical
Reconstructing evolutionary transitions between the various types of symmetry requires rigorous assessment of primary homologies through comparative anatomy and their interpretation under a phylogenetic framework. The phylogenetic distribution of adult symmetry types onto the animal tree is presented on Fig. 6A (probable ancestral character state for each terminal taxon indicated on tip of the branches; see legend for justification). I use here as a working framework a consensus tree derived from rRNA molecular phylogenies [19,32,82,107,120,130,158,190], although in fact basal metazoan phylogeny remains uncertain, since various data sets have provide inconclusive or conflicting results [49,64,168]. I have decided to leave apart the placozoan Trichoplax for its position is totally unresolved (see for example [48]) and its type of symmetry is ambiguous (asymmetrical or cylindrical; see above).
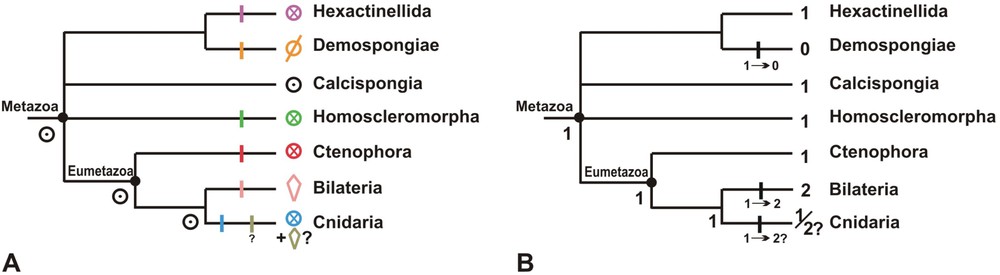
Scenarios for the evolution of the types of symmetry (A) and of the axial properties (B), on a “consensus” phylogenetic tree from rRNA molecular phylogenies. Controversial aspects of the phylogeny include the status of sponges (monophyly vs. paraphyly) and the position of the ctenophores. See text for explanations on scenarios. Symbols: ∅: asymmetry; ⊙: cylindrical symmetry; ⊗: radial symmetry; ◊: bilateral symmetry.
Scénarios d'évolution des types de symétrie (A) et des propriétés axiales (B), sur un arbre phylogénétique « consensus » issu des travaux de phylogénie moléculaire basés sur les ARNr. Les aspects les plus discutés de la phylogénie sont le statut des éponges (monophylie ou paraphylie) et la position des cténaires. Voir le texte pour les explications sur ces scénarios. Symboles : ∅ : asymétrie ; ⊙ : symétrie cylindrique ; ⊗ : symétrie radiaire ; ◊ : symétrie bilatérale.
The evolution of symmetry types cannot be directly inferred from parsimony-based character optimisation because of the lack of phylogenetic resolution combined with high number of character states and high level of homoplasy. I will nevertheless try to propose an evolutionary scenario (depicted in Fig. 6A), but the reasoning will be indirect and rather speculative. Instead of directly optimising the types of symmetry, it is simpler to start by considering the axial properties of the adult body, formalised into a three-state character with state 0 corresponding to asymmetry, state 1 to the presence of a single axis of symmetry and body polarity (monaxonic heteropolar organisation), and state 2 to the presence of two body polarity axes (bilaterality) (Fig. 6B). Here, parsimony optimisation favours state 1 on the common node of Metazoa (with a minimum of 3 steps, against 4 steps for states 0 or 2 on the same node). Thus, with reservations due to uncertainty on the phylogeny and to the weak deviation in step numbers between competing hypotheses, this simple reasoning indicates that the last common ancestor of Metazoa was probably symmetrical around a single polarity axis, rather than asymmetrical as postulated in the “conventional scenario”.
Accordingly, the adult asymmetrical organisation observed in most demosponges is probably derived rather than ancestral. Demosponges are just one out of four major extant sponge lineages [20], and the other three (hexactinellids, calcisponges and homoscleromorphs) have prevalent adult cylindrical or radial symmetry (Figs. 4A, 5A–D). Some calcisponges also are asymmetrical (e.g. Leuconia), but this is clearly a derived situation within the Calcispongia, with respect to a plesiomorphic monaxonic heteropolar adult organisation [118,120,121]. Of particular significance is the radial symmetry of homoscleromorph sponges (Fig. 5C–D) [67,68,93,176], a group recently separated from demosponges on the basis on molecular, histological and embryological characters [20,68,180]. The extinct sponge lineage Archaeocyatha (of uncertain affinity with respect to extant sponge taxa) is also characterised by a clear radial architecture. Thus, the common idea that sponges, supposedly the “most primitive” animals, would be devoid of any defined adult body symmetry and/or polarity axis [6,27,70,71,94,97,157,169,173,193] (concerning Ref. [27], see their matrix, pp. 894–895) must be vigourously refuted, as it is entirely attributable to taxonomic bias (the demosponges representing 95% of living sponges species) and typological reasoning (demosponge morphology having been taken as “typical” for sponges).
6.2 The metazoan ancestor was probably cylindrically symmetrical
If the common metazoan ancestor was polarised along a single symmetry axis (state 1, Fig. 6B), that leaves two possibilities for the ancestral type of symmetry: cylindrical or radial. The crucial question is now whether the radially symmetrical anatomies (with repeated structures around the axis) occurring in various basal metazoan lineages are homologous or not. I argue below from comparative anatomy that they are clearly not. The proposed hypothesis of multiple convergences implies that each kind of radial symmetry actually represents a distinct character state, explaining why I have used different colours on Fig. 6A.
The multiradiate symmetries of hexactinellids, syconoid calcisponges and homoscleromorphs are certainly convergent. Molecular phylogeny suggested that the ancestral aquiferous system of calcisponges was asconoid (cylindrical symmetry), a derived syconoid aquiferous system (radial symmetry) being acquired several times independently within the clade [118,120,121] (but see criticism in Dohrmann et al. [55]). Hence, homology with the syconoid-like organisation observed in homoscleromorphs is unlikely. The multiradiate organisation of most hexactinellids entirely depends on the architecture of their skeleton (Fig. 5A), which itself results from the particular geometry and arrangement of their unique hexactine siliceous spicules [5,189]. These arguments also imply that instances of multiradiality in sponges are absolutely not homologous with radial anatomies occurring in ctenophores or cnidarians. Consistently, the strict determination and low value of symmetry plane number in ctenophores and cnidarians (with the exception of hexacorallian anthozoans) departs fundamentally from the high and indefinite number which characterises multiradial symmetry in sponges. The only reported instance of tetraradial symmetry in sponges, the regular disposition of four particular cells (“cellules en croix”) in the flagellated anterior pole of the calcisponge amphiblastula larva [59,188], has clearly nothing to do with the tetraradial symmetries of ctenophores or cnidarians which involve multicellular structures.
Convergence of the radial symmetry between ctenophores and cnidarians is equally likely, because their repeated structures are clearly not homologous. The symmetry of ctenophores (Fig. 5E) is in fact a unique combination of octoradial (eight comb rows, eight meridional canals), tetraradial (four balancers – mechanoreceptor structures supporting the statolith, within the apical sensory organ – four pairs of comb rows, four interradial canals) and prevalent biradial symmetry (e.g. one tentacle pair, one pair of polar fields, a flattened pharynx), the latter being disrupted by the oblique position of the two anal pores. By contrast, in the polyp, the probable ancestral body form of the Cnidaria [8,22,33,34,122], the only repeated structures are the tentacles (arranged in a crown around the mouth) and the mesenteries (or septae), endodermal infoldings of the gastral cavity wall. The latter occur in anthozoan (Fig. 7) and scyphozoan polyps, and are supposed to be secondarily lost in the simplified polyps of Hydrozoa and Cubozoa [122]. Thus, ctenophore radially repeated structures do not exist in the cnidarian body plan, and reciprocally, with the exception of tentacles. However, tentacles are clearly non-homologous between these two phyla, as indicated by their totally different position in the body plan, and by their differing structures and ontogenies [90].
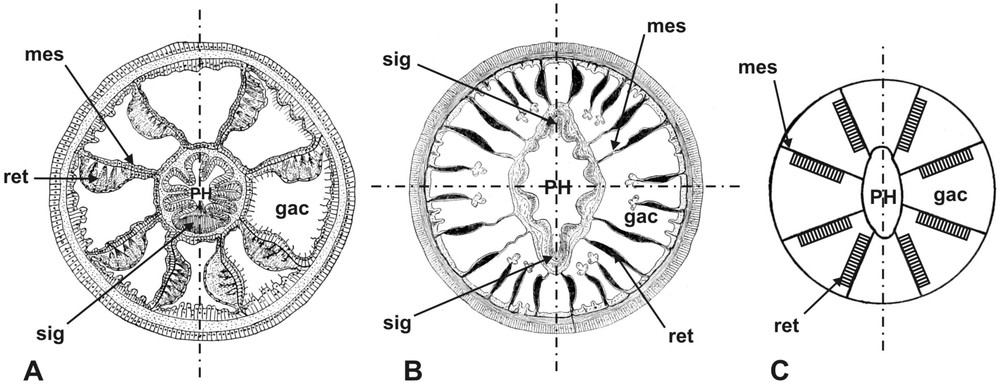
Symmetry in anthozoan cnidarians, with planes of symmetry indicated by the dashed lines. All sections are at the level of the pharynx. A: transverse section in an octocoral polyp (Octocorallia). B: transverse section in a sea anemone (Sagartiidae, Actiniaria, Hexacorallia). C: schematic transverse section in the Edwardsia larval stage of a sea anemone (gac: gastral cavity; mes: mesentery; ret: retractor muscles; PH: pharynx; sig: siphonoglyph) (after [98]).
Les symétries chez les cnidaires anthozoaires. Les plans de symétrie sont indiqués par les traits en pointillés. Toutes les coupes sont au niveau du pharynx. A : coupe transversale dans un polype d'octocoralliaire. B : coupe transversale dans une anémone de mer (Sagartiidae, Actiniaria, Hexacorallia). C : coupe transversale schématique du stade Edwardsia d'une anémone de mer (gac : cavité gastrique ; mes : mésentère ; ret : muscle rétracteur ; PH : pharynx ; sig : siphonoglyphe) (d'après [98]).
In strict parsimony terms, “state 1” (monaxonic heteropolar organisation) present in the common metazoan ancestor could thus correspond to any of the various kinds of radial symmetry observed in non-bilaterian lineages, or to cylindrical symmetry. However, that the latter is the ancestral state is strongly supported by ontogeny, since the cylindrically symmetrical stage through which all metazoans (including the bilaterians) pass during their ontogeny is easily interpreted as an instance of recapitulation. This idea is extremely classical, since in the haeckelian theory of metazoan origin [88], the Gastraea was postulated to be the cylindrically symmetrical ancestor of all metazoans (following a spherical stage called the Blastaea). The primitiveness among adult metazoan body plans of a monaxonic (rather than asymmetrical) structure has always retained a minority of supporters (e.g. Beklemishev [15]). Admitting cylindrical symmetry as ancestral, it can be noticed that the only extant metazoans retaining this organisation as adults are the asconoid calcisponges (e.g. genera Clathrina, Soleneiscus, Leucosolenia…), and as such, these sponges should receive special attention in future comparative studies of polarity-generating molecular mechanisms. By contrast, the partial cylindrical symmetry of polyps in hydrozoan and cubozoan cnidarians (at the body column level) probably results from a reversion (i.e. loss of the mesenteries [122]).
6.3 Anatomical comparison of the bilateral symmetry in anthozoans and bilaterians
Bilaterality is clearly a derived type of symmetry since it occurs only in the closely related Cnidaria and Bilateria. We thus need to compare the nature of bilaterality in these two clades to evaluate if it could have been inherited from their common ancestor, as believed by a few morphologists [86,99,193]. I will discuss recent arguments from developmental gene data later on in the following section. In-depth consideration of the anatomical, biological and phylogenetic significance of bilaterality among anthozoan cnidarians is particularly crucial, because failure to do so in the recent evo-devo literature has seriously hampered the interpretation of molecular data. Exceptional instances of bilaterality in hydrozoans (see Section 4) will not be discussed here, because they are clearly derived (several times independently) from within this class and thereby convergent with the bilaterality of the Bilateria.
Although the bilateral symmetry of anthozoans has been widely popularised through recent molecular studies on a sea anemone, bilaterality is considerably more evident in the polyp anatomy of the anthozoan order Octocorallia (Fig. 7A) [98]. In an octocoral polyp, two types of anatomical structures are affected by bilateral symmetry: the pharynx (an ectodermal invagination leading to the gastral cavity) and the mesenteries (Fig. 7A). The flattened pharynx is bilaterally symmetrical, due to the presence of a single ciliated groove or siphonoglyph (Fig. 7A), driving a water current into the gastral cavity. The eight mesenteries are globally arranged in an octoradial pattern, but they differ with each other in their constitutive elements, and identical mesenteries are bilaterally opposed. In particular, each mesentery bears on one of its faces a retractor muscle, of mesodermal origin [179], and the relative distribution of the eight retractor muscles is bilaterally symmetrical (Fig. 7A). In addition, in transverse section below the pharynx (not shown), the two mesenteries located on the side opposite to the siphonoglyph differ from the other in lacking gonads and in having ciliated ectodermal free edges (mesenterial filaments) serving to create an upward water current, instead of the cnidoglandular endodermal mesenterial filaments (whose function is digestive) associated with the six remaining mesenteries [98]. The octocorallian polyp is thus truely bilateral. Its second polarity axis, orthogonal to the main oral–aboral axis, is called the directive axis.
By contrast, in most adult sea anemones (Actiniaria), the disposition of mesenteries is absolutely radial (6n) with no trace of bilateral symmetry (Fig. 7B) and because there are two siphonoglyphs, also the pharynx is biradial. Hence, these sea anemones (e.g. Anemonia, Sagartia, Metridium…) are not at all bilateral. The same holds true for corals (Scleractinia and the akin “naked corals” Corallimorpharia), in which siphonoglyphs are absent. In these anthozoans, bilateral symmetry is only transiently apparent during development, in the disposition of the eight retractor muscles at the “Edwardsia” larval stage (Fig. 7C), before the production of additional mesenteries. However, several sea anemone lineages have diverged ecologically by adopting a burrowing life style in sediments, and correlatively their ontogeny is characterised by paedomorphosis, i.e. as adults they look like Edwardsia larvae [43]. The laboratory model Nematostella vectensis (family Edwardsiidae), whose complete genome has been sequenced, is such a highly derived sea anemone, explaining why throughout its life it retains eight mesenteries (and the concomitant bilaterality of retractor muscles), and a single siphonoglyph (hence bilaterality of the pharynx). Through paedomorphosis, the Edwardsiidae have reverted to more plesiomorphic character states found in other anthozoans such as octocorallians.
The phylogeny of Anthozoa is still largely unresolved, but recent studies based on various kinds of data sets tend to resolve the Octocorallia as the sister-group of other anthozoans (Hexacorallia), the latter comprising the Ceriantharia and a clade grouping all other taxa (Antipatharia, Zoanthidea, Actiniaria, Madreporaria) (France et al. [76] with mitochondrial 16S, Berntson et al. [18] with 18S rRNA, Won et al. [194] with morphology, Daly et al. [44] with combined morphology, 18S, 28S and 16S; reviewed in Daly et al. [45]). Since Octocorallia, Ceriantharia and Zoanthidea have a single siphonoglyph, a bilateral pharynx was probably present in the common ancestor of Anthozoa, while pharynx biradiality evolved secondarily through siphonoglyph loss in Madreporaria (= Scleractinia + Corallimorpharia) or through the addition of a second siphonoglyph in Antipatharia and Actiniaria. Furthermore, mesenteries exhibit some level of bilaterality at least during ontogeny in all hexacorallian lineages [15,17,98]. Thus, bilateral symmetry was clearly present in the last common ancestor of Anthozoa, or even (perhaps) in the common ancestor of the Cnidaria since a recent analysis of mitochondrial genomes obtained the paraphyly of anthozoans with respect to the other cnidarians (Medusozoa) [105], in conflict with rRNA phylogenies supporting their monophyly [18,33,76,105,151].
However, the bilateral symmetries of Anthozoa and Bilateria are in no way comparable. The bilaterality of anthozoans is clearly superimposed on a fundamentally radial anatomy, of which bilaterians show no trace. Whereas in the latter, the whole body plan is affected by bilaterality (general body shape, all germ-layers and virtually all organ systems), in anthozoans, only a restricted set of internal structures (pharynx and mesenteries) are bilateral, whereas the rest of the body (particularly the external morphology) is absolutely unaffected. Most significantly, all anthozoan bilateral structures are evolutionary acquisitions (synapomorphies) of the Anthozoa or Cnidaria, with no counterpart in bilaterian body plans. The mesenteries are a synapomorphy of the Cnidaria [122], while the mesentery retractor muscles and the ectodermal pharynx with a siphonoglyph are synapomorphies of the Anthozoa [122] (or of the Cnidaria, if anthozoans are paraphyletic [105]). Thus, the common ancestor of Cnidaria and Bilateria lacked all the structures that are bilaterally disposed in the Anthozoa. Also the biological significance of bilaterality is totally different between Bilateria and Anthozoa. In anthozoans, bilaterality has nothing to do with directional locomotion but is linked to the canalisation of water currents within internal cavities [15,98] and to the mode of mesentery production [17]. It is hard to imagine how this anthozoan-type internal bilaterality could have turned to become advantageous for unidirectional locomotion through exaptation in a bilaterian ancestor, as recently proposed [72]. In conclusion, there is compelling evidence for the convergent acquisition of bilaterality between the anthozoans and the bilaterians – actually the most common point of view among morphologists, including Hyman [98] and Beklemishev [15].
6.4 Conclusion: a scenario for the evolution of symmetry types
From the preceding overview of symmetry types across basal metazoan lineages, it is clear that the “conventional scenario” (a stepwise succession of asymmetry, radiality and bilaterality) is untenable. The most likely scenario places cylindrical symmetry as the ancestral state, while asymmetry, radial symmetry and bilateral symmetry (and exceptionnally, spherical symmetry) are derived types, each evolved several times independently, in the terminal branches, or within phyla (Fig. 6A). Multiple convergences as implied by the proposed scenario are coherent with my previous suggestion that important shifts in body symmetry did not necessitate any particular genetic or developmental innovation (thus, they could have occurred easily and repeatedly), and with the multiplicity of potential functionalities offered by each one of the symmetry types. A further notable implication of this scenario is that the multicellular ancestors of the Bilateria never passed through an asymmetrical or a radial type of symmetry.
7 Developmental genes and the homology of polarity axes: the evo-devo perspective
The comparative anatomy approach outlined in the previous section suffers from an inherent limitation stemming from the impossibility of uncoupling symmetry or polarity from the anatomical structures that reflect them. However, anatomical structures distributed along an axis inherited from a common ancestor may diverge between lineages to the extent that homology of the axis itself is no longer recognisable. This argument for example runs against my previous arguments for convergence of radial anatomies (structures may be convergent but the angular axis homologous). Reciprocally, I have implicitely assumed above the homology of the main body axis across all lineages, but in fact, this homology is not decisively supported by comparative anatomy and embryology.
To circumvent this problem, we need characters that are at the same time amenable to the application of homology criterions and intrinsically linked to polarity, not to particular anatomical structures. Molecular mechanisms involved in the establishment and maintenance of polarities have these properties, with the underlying expectation that their conservation (in terms of gene orthologies, relative distribution of their expression territories, and functional interactions) should reflect shared ancestry (homology) of the corresponding body axes. This approach has been a successful evo-devo research program since the early 1990's [81]. I will thus examine successively three major themes emerging from the recent evo-devo literature: the proposed homology between the anthozoan directive axis and the bilaterian DV axis; the correspondence between the cnidarian oral–aboral axis and the bilaterian AP axis based on the expression of Hox genes and other transcription factors; and the molecular nature of the hypothesised ancestral body polarity axis of the Metazoa.
7.1 Do molecular data support homology between the bilaterian DV axis and the anthozoan directive axis?
In distantly related bilaterians, the generation of DV polarity involves a conserved protein network which comprises, among others, secreted molecules of the BMP family (e.g. decapentaplegic = dpp in Drosophila and its orthologue BMP4 in vertebrates) and their antagonist secreted ligands (e.g. short gastrulation = sog in Drosophila and its orthologue chordin in vertebrates) (Fig. 8A) [51,52,80]. This “BMP-chordin axis” functioned in the common ancestor of Bilateria, as supported by evidence not only from vertebrates and arthropods, but also from hemichordates, echinoderms and annelids [50,57,115]. Curiously, the polarity of DV signalling molecules is inverted in chordates with respect to other bilaterians (e.g. arthropods, but also annelids and hemichordates), suggesting that an exclusive ancestor of chordates turned upside down (the DV inversion hypothesis [7,51,115]).
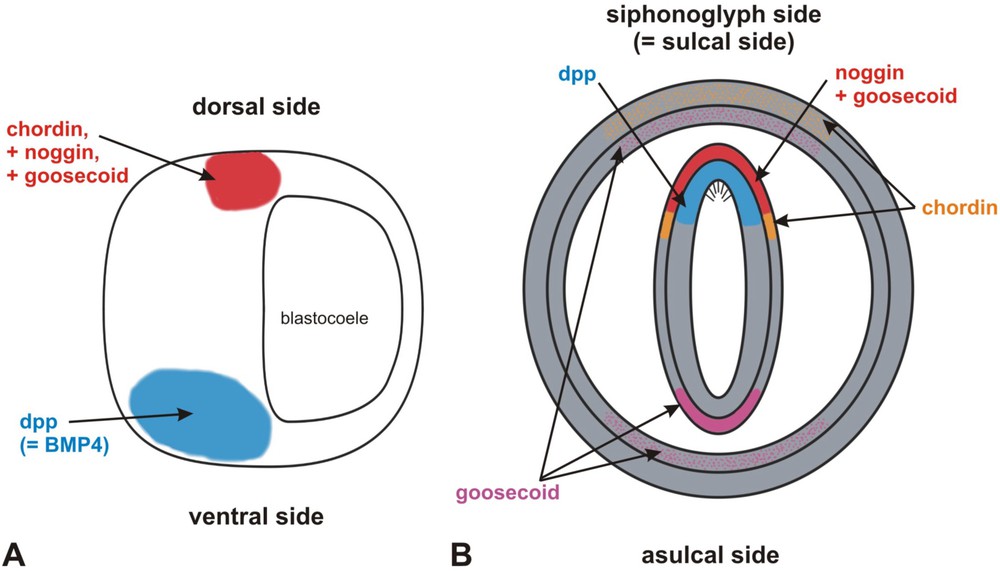
Comparison of the expression patterns of four of the genes involved in dorso-ventral axis patterning in the Bilateria and of their orthologues in the sea anemone Nematostella. A: gene expressino patterns in an amphibian gastrula. B: gene expression patterns in the sea anemone, in transverse section at the level of the pharynx. Dots indicate a weaker level of expression (A: after [80]; B: after [127]).
Comparaison des patrons d'expression de quatre des gènes impliqués dans le patterning de l'axe dorso-ventral chez les Bilateria, et de leurs homologues chez l'anémone de mer Nematostella. A : patrons d'expression chez une gastrula d'amphibien. B : patrons d'expression chez l'anémone, en coupe transversale au niveau du pharynx. Les pointillés matérialisent un niveau d'expression plus faible (A : d'après [80] ; B : d'après [127]).
In the planula larva of the sea anemone Nematostella vectensis, homologues of these bilaterian DV signalling molecules (e.g. dpp, noggin, chordin) and transcription factors (e.g. goosecoid) display restricted expression territories along the directive axis (Fig. 8B) [75,127,128,164]. The dpp homologue is likewise expressed asymmetrically in the coral Acropora [92]. According to Matus et al. [127], “the fact that NvChordin, NvNoggin1, NvGsc and NvDpp all are expressed asymmetrically along the directive axis at some point in the development of the juvenile body suggests that the cnidarian directive axis might be homologous to the bilaterian DV axis”. Thus, bilaterality would have evolved in a common ancestor of Cnidaria and Bilateria, and have been secondarily lost in non-bilateral cnidarians (the Medusozoa) [12,71,72,75,127,171]. This hypothesis is at odds with indications from comparative anatomy that bilaterality in these two lineages is certainly convergent (see preceding section).
However, these asymmetric gene expression patterns are not by themselves sufficient to support axis homology. A strict application of the principle of connexions to the gene expression patterns would expect orthologues of dorsal vs. ventral bilaterian molecules to be consistently expressed at one extremity and the other of the anthozoan directive axis, which does not happen to be the case (Fig. 8A, B). In chordates, dpp-BMP4 is mostly expressed ventrally, and the antagonists chordin and noggin are expressed on the dorsal side (Fig. 8A), while in the sea anemone, the three molecules are expressed on the siphonoglyph side of the directive axis [127,164], albeit in distinct germ layers (Fig. 8B). The transcription factor goosecoid, active in the dorsal organiser of chordates [80] (Fig. 8A), is expressed on both sides of the directive axis in the sea anemone (Fig. 8B). To these considerations it can be objected that signalling molecules operate at some distance from their transcription site, and that interacting diffusing factors (activators vs. inhibitors) may have differing diffusion capacities [134]. Thus, antagonist interaction between dpp and chordin proteins could be involved in the specification of the directive axis, even though their mRNAs locate on the same side. Only through in vivo functional experiments will the exact link between these molecules and directive axis polarity be understood. Injection experiments of the dpp and chordin cnidarian homologues in zebrafish [164,165] have demonstrated that the molecular antagonism is operating in the sea anemone, but these data do not indicate whether this mechanism is involved in generating the directive axis, or instead governs smaller scale polarities, such as for example the specification of germ layers on the siphonoglyph side [128].
Even if dpp signalling were actually specifying the sea anemone directive axis, this would not represent a strong case for homology with the bilaterian DV axis. Because morphologically the anthozoans are clearly bilateral, signalling molecules must operate to establish the directive vs. oral/aboral axis. The dpp system, present in the common cnidarian–bilaterian ancestor, could well have been adopted independently in these two lineages for creating a second axis (a phenomenon known as co-option). In conclusion, since available molecular data on cnidarian homologues of bilaterian DV genes are not compelling in favour of bilaterality being inherited from a cnidarian–bilaterian ancestor, convergence as supported by comparative anatomy clearly remains the most satisfying hypothesis.
7.2 Hox genes and the correspondence between the cnidarian oral–aboral axis and the bilaterian AP axis
The possibility remains that at least the cnidarian oral–aboral and the bilaterian AP axes would be homologous. Although admitted by most classical zoologists, this homology is not evident on anatomical grounds. The cnidarian oral region offers no resemblance with a bilaterian head (e.g. there is no brain), apart from the presence of the mouth, and cnidarians lack the anus which terminates the body of most bilaterians. Even if the axes were homologous, the cnidarian oral pole could correspond either to the anterior or to the posterior pole of the Bilateria. In the planula larva of the Cnidaria, the future aboral pole is generally located anteriorly during swimming. In some species this pole bears a sensory organ, which in the sea anemone expresses components of the FGF pathway, similarly to the anteriorly located apical organs associated with the brain of bilaterian planktonic larvae [166]. These observations favour the counter-intuitive hypothesis that the cnidarian counterpart of the bilaterian head would be the aboral and not the oral pole.
The alternative view, that the cnidarian oral pole corresponds to the bilaterian head, has been much more popularised in recent years, principally from expression data on the sea anemone homologues of the bilaterian Hox genes (in addition to earlier questionable arguments based on the expression of bilaterian “head specific” genes in the Hydra hypostome organiser [77,138]). Among the Bilateria, Hox genes have a well-known conserved role in AP patterning [80,156]. These homeobox genes are organised in a genomic cluster and in most investigated bilaterians, they are expressed along the AP axis in a sequential order which corresponds to their respective location within the cluster (a property called spatial colinearity). The common ancestor of Bilateria certainly used a “Hox code” to pattern its AP axis, although the system underwent many substantial modifications within the various bilaterian lineages [60]. Hox genes are not involved in the early establishment of the AP axis, a process involving various maternally localised determinants and signalling molecules (see later), but they act in controlling the correct positioning of structures along an already established axis.
The Hox gene family by itself is not an innovation of the Bilateria. Cnidarians have clear Hox homologues (these are called Anthox in anthozoan cnidarians and Cnox in hydrozoan cnidarians) [75,79,172]. Trichoplax lacks any Hox but possesses a ParaHox gene (called Trox2) [100,141] and since ParaHox is the supposed Hox sister cluster [78], the absence of Hox in Trichoplax could well be secondary. Hox and ParaHox genes are absent from the Amphimedon demosponge genome [110] and attempts to recover them from other demosponge and calcisponge taxa [119,167,178] have failed. There is, however, a debate in the literature to determine if the absence of Hox/ParaHox in sponges is ancestral [110] or derived [159]. Similarly, tentatives to identify Hox/ParaHox members in ctenophores have remained until now unsuccessful (but definitive proof of their absence is waiting for the availability of a complete genome). Thus, even if this requires confirmation, the Hox/ParaHox family could be a synapomorphy of (Trichoplax + Cnidaria + Bilateria).
Whereas the expression of Hox homologues in the Hydra polyp did not evoke a function in oral–aboral axis patterning [79], the expression of Anthox genes in the sea anemone Nematostella was claimed to support the fascinating conclusion that Hox-based patterning was inherited from the cnidarian–bilaterian ancestor and that the oral–aboral axis of Cnidaria was homologous to the AP axis of Bilateria, with the polyp oral extremity corresponding to the bilaterian head [75,172]. However, as for the DV gene data discussed earlier, these far-reaching evolutionary interpretations were elaborated on limited evidence. Of the 7 studied Anthox genes, only two showed restricted expression along the oral–aboral axis. The Anthox6 gene, an orthologue of the bilaterian anterior Hox gene Hox1/labial, was expressed at the oral pole. The Anthox1 gene, a putative orthologue of bilaterian posterior genes (Hox9-13/AbdB/Post), was localised at the opposite aboral extremity. All other investigated Hox homologues were expressed all along the body column (but they were localised along the directive axis) [75,172].
In conflict with these early interpretations of the Nematostella Anthox data, recently accumulated evidence strongly argues against a conserved role for Hox homologues in patterning the oral–aboral axis of Cnidaria in a way similar to the AP patterning Hox system in the Bilateria. First, orthologies between cnidarian genes and the various genes of the bilaterian Hox cluster (and of its supposed sister cluster ParaHox) are in general unclear. Among published Hox gene phylogenies (e.g. [31,79,104,172]), the only points of agreement are the existence of bona fide cnidarian orthologues of bilaterian anterior Hox genes, and the absence of cnidarian orthologues of bilaterian median genes. Other aspects are more controversial and there are conflicting views concerning the early history of the Hox/ParaHox genes (e.g. [31,69,73,78]). The second major problem is the absence of a genomic cluster grouping Hox homologues in the Nematostella genome. Anthox genes are dispersed among the genome (corresponding to an “atomised” cluster in the classification by Duboule [60]), apart from the probable ancient synteny represented by the association between eve (a “Hox like” homeobox gene) and the “anterior” Anthox genes (Anthox6, Anthox7, Anthox8a, Anthox8b). Although possibly derived, this absence of Hox clustering in Nematostella implies de facto an absence of spatial colinearity of gene expression.
Even more problematic is the strong variability of Hox gene expression along the oral–aboral axis among cnidarian lineages. Whereas the Hox1/labial “anterior Hox” homologue Anthox6 gene is orally expressed in Nematostella, its orthologue Cnox1 in the hydrozoan Podocoryne is expressed in opposite orientation [104,196]. Considerable variation among cnidarian taxa is also observed for the ParaHox gene Cnox2/Gsx [74]. Curiously, in cnidarians we are faced with the opposite situation to that observed in bilaterians: while among the later, Hox gene expression along the AP axis is conserved in spite of a tremendous disparity of body plans, their cnidarian orthologues have highly plastic expression territories along the oral/aboral axis, in animals that share the same basic body plan.
In conclusion, contrary to earlier and persisting claims [75,172], current evidence does not support a conserved role for cnidarian Hox homologues in patterning the oral/aboral axis, in a way similar to AP axis patterning in the Bilateria [104]. Although the situation observed for Hox genes in cnidarians could be derived, currently the most parsimonious interpretation is that colinearity along the AP axis was a bilaterian innovation [104], possibly linked to the novel requirements imposed by directional locomotion on the antero-posterior patterning of the central nervous system [53]. An additional (somewhat disappointing) conclusion is that Hox genes do not tell us which extremity of a cnidarian polyp is homologous to the bilaterian head, if such homology exists. Recent expression studies of cnidarian Otx and emx, two transcription factors involved in anterior patterning of the central nervous system in bilaterians, gave similarly unconclusive results with respect to cnidarian/bilaterian “head” homology [103,129].
The general trend is that homologues of transcriptions factors with conserved patterning functions along the polarity axes of the Bilateria do not show consistent localisations along the cnidarian oral–aboral axis. In addition, transcription factors belonging to both the DV and AP patterning systems of bilaterians were found expressed in regionally restricted patterns along the cnidarian oral/aboral axis [103], leading to the suggestion that the latter might be homologous to both polarity axes of the Bilateria. The AP and DV axes would thus have originated from a sort of duplication of a single ancestral axis, with subfunctionalisation of the corresponding signalling and patterning molecules between both novel axes [95,103,132,134,165].
7.3 Wnt signalling molecules and the ancestral polarity axis
Hox genes and other transcription factors are certainly not the best candidates if we wish to uncover deep correspondences between the polarity axes of basal metazoan lineages, since, as noted in a previous section, the earliest cell fate differences appearing in embryos are mediated by secreted ligands turning on signalling pathways, while transcription factors are acting downstream. The search for ancient polarity axis generating molecular mechanisms has recently focalised on the Wnt pathway [84,112].
It has become apparent in recent years that in the Bilateria, Wnt signalling plays a conserved and probably ancestral role in establishing and patterning the AP axis during early development [96]. In vertebrates, Wnt signalling has an early involvement in the formation of the dorsal organiser, and later acts to posteriorise during gastrulation [96,133,134]. Data from vertebrates, echinoderms and amphioxus supports the conservation among deuterostomes of a “posterior centre” secreting Wnt proteins, in addition to expressing a conserved cassette of regulatory molecules such as genes of the Notch-Delta pathway, and the transcription factors Brachyury and Caudal [36,96]. The conservation may extend to the Bilateria since various protostomes show posterior Wnt expression [177].
Recent studies on various cnidarians have highlighted the role of Wnt signalling in establishing and patterning the cnidarian oral–aboral axis. In the adult polyp of Hydra, axial polarity is constitutively under the control of the hypostome organiser, in the oral region [24]. The Wnt “canonical” pathway is active in this “head organiser”, as demonstrated by the localised expression of HyWnt and of another components of the pathway (HyTcf) in this region, by the higher level of nuclear β-catenin in the hypostome than in cells of the body column, and by the formation of ectopic oral structures following treatments with molecules (DAG, LiCl, alsterpaullone) that activate the canonical pathway [25,95,145]. Comparable experiments in another hydrozoan, Hydractinia, gave similar results [146,147,161].
Wnt signalling is also involved in the early establishment of the oral–aboral axis during embryogenesis in cnidarians. In the hydrozoan Clytia hemisphaerica, two (maternally inherited) oppositely localised Wnt receptors (Frizzled) govern oral–aboral polarity, as indicated by inhibition and over-expression experiments [139]. The Wnt canonical pathway is activated at the animal pole, the future blastopore and mouth region, as demonstrated by β-catenin detection assays. Experimental data likewise indicated activation of the Wnt canonical pathway at the oral pole in the other hydrozoan Hydractinia [161] and in the sea anemone Nematostella [113,192]. In addition, this region also expresses Brachyury [175], suggesting that the same cassette is acting at the cnidarian oral pole and in the bilaterian “posterior centre”. We are thus reverting to the hypothesis, consistent with the swimming direction of the planula, that the cnidarian oral extremity is homologous to the bilaterian posterior pole, in contradiction with suggestions based on Hox expression in the sea anemone [75,172] but in consistence with earlier proposals prompted by gene expression data in Hydra [131,133].
Not only are the Wnt mediating the early establishment of the cnidarian oral–aboral axis, but there is also some evidence that they are involved in later patterning along this axis. The sea anemone genome contains 12 different Wnt genes, belonging to 11 of the 12 families previously identified in bilaterian genomes, indicating that Wnt diversification predated the cnidarian–bilaterian divergence [84,109,112]. In the sea anemone, most of these Wnt genes are first expressed around the blastopore, and later on at the planula stage they become expressed along the oral–aboral axis, in overlapping domains, either in the ectoderm or in the endoderm, depending on the genes (Fig. 9) [109]. Wnt genes in Clytia embryos are also expressed in distinct nested territories along the developing oral–aboral axis, although there is no obvious correspondence between the territories of orthologous genes with Nematostella [140]. The global pattern of Wnt gene expression along the cnidarian oral–aboral is somewhat reminiscent of Hox gene expression in the Bilateria and has suggested the implication of a “Wnt code” in oral–aboral axis patterning in cnidarians [84,109,112], although functional data are required for explaining how this Wnt code could work.

Overlapping expression domains of eight Wnt genes, in staggered arrays along the oral–aboral axis, in a Nematostella vectensis planula. Depending on the genes, expression is observed in the ectoderm (above) or in the endoderm (below) (after [109]).
Les domaines d'expression de huit gènes Wnt sont emboîtés le long de l'axe oral–aboral, dans la planula de Nematostella vectensis. Selon les gènes, l'expression est restreinte à l'ectoderme (en haut du dessin) ou à l'endoderme (en-dessous du dessin) (d'après [109]).
The Nematostella data also indicates that, in addition to axial polarity, Wnt signalling is necessary for gastrulation and the specification of germ layers [109,113,192]. In fact, axial differentiation, gastrulation and germ-layer specification are concomitant and highly interdependent processes – meaning, in other words, that the specification of both spatial coordinate systems (axial and radial) previously defined for cylindrical symmetry (Fig. 1) is tightly coupled. In bilaterians, Wnt signalling determines not only axial differences in cell fate, but also gastrulation movements and germ-layer specification. For example, in vertebrates and echinoderms, the canonical Wnt pathway is required for the specification of the endomesoderm [36]. In chordates, the coordination of cell movements during gastrulation is under the control of the “planar cell polarity” (PCP) Wnt pathway; and the Brachyury transcription factor has well-known roles in the control of gastrulation and mesoderm specification [183]. The reported effect of Frizzled1 inactivation on cilia alignment and cell movement in the Clytia embryo [139] suggests that Wnt signalling through the PCP pathway may similarly act in cnidarian gastrulation. That Wnt signalling governs both axial cell fate differences and gastrulation movements leaves opened the question of which of these two roles is the most ancestral.
The origin of Wnt involvement in axial polarity has been recently pushed even earlier in time by expression data in the sponge Amphimedon, showing localised expression of Wnt and genes at opposite poles of the sponge embryo and larva [2]. Wnt expression becomes localised at the future posterior pole of the embryo (with respect to the swimming direction of the larva) before any morphological polarity is detectable. Thus, a signalling centre similar to the bilaterian posterior centre and the cnidarian blastoporal centre might act on the sponge larva posterior pole. However, functional experiments and information on other genes of the cassette (e.g. Notch, Brachyury), as well as data on other sponge taxa, are requested for confirming these still preliminary conclusions.
Thus, with reservations due to the absence of Wnt data in ctenophores and still preliminary data in sponges, Wnt signalling could be the most ancient mechanism for establishing early cell fate differences along the main body axis [112,171] (but for a more sceptical point of view see Primus and Freeman [162]). A correlative suggestion is that the main body axes of sponges, cnidarians and bilaterians are probably homologous. It is furthermore tempting to speculate about a possible correspondence between the posterior pole of sponge and cnidarian larvae (and thus the adult sponge apical extremity and the polyp oral extremity) and the rear end of bilaterian animals, based on the position of the Wnt centre. In any case, it is clear that the acquisition of Wnt genes in an exclusive ancestor of the Metazoa (they are absent from non-metazoan eukaryote genomes) was a decisive event with respect to the later evolution of animal body plans.
8 Conclusion
A coherent picture of the early evolution of symmetry and polarity is emerging from the synthesis of data from comparative anatomy, phylogeny and evo-devo. The last common ancestor of Metazoa was probably cylindrically symmetrical, with a concomitant axial polarity generated by early embryonic signalling involving the Wnt pathway. The wide range of signalling molecules and other developmental genes already existing in the genome of this ancestor endowed its descendants with enormous potential for evolving simpler or more complex new anatomies (e.g. various types of radially repeated structures, bilateral symmetry, asymmetry, etc.). Changes in symmetry type occurred repeatedly, with architectural constraint and adaptive value (with respect to environmental parameters) as the main constraining forces, providing a wonderful example of evolutionary “tinkering”.
These suggestions clearly need further testing in the future. Progress in phylogenetic reconstruction of basal metazoan relationships, notably through the phylogenomic approach [160] are warranted, to clarify the early evolution of key anatomical characters linked to symmetry and polarity. Future evo-devo prospects include investigations on early polarity-generating molecular mechanisms in the various sponge lineages, the ctenophores and Trichoplax, and comparative studies, particularly in ctenophores and cnidarians, on the molecular mechanisms responsible for the repetition of parts around an axis (radial symmetry in the strict sense), still a terra incognita.
Recent advances on the characterisation of molecules involved in the establishment and maintenance of animal polarities, and their comparison across lineages, have deeply renewed our approach to the problem of homology between axes of distantly related animals, by concretising abstract notions of promorphology (e.g. geometrical axes and coordinates) or experimental embryology concepts (e.g. morphogen, morphogenetic field) into molecular actors and operations. However, there are also potential pitfalls in this area. Notably, because genes and gene networks are frequently co-opted for novel functions during evolution, there is no simple relation between similar genetic mechanisms and anatomical homology, a problematic that has been extensively dealt with elsewhere [54,150,170,182]. In particular, signalling pathways should not be taken as infallible markers of axis homology, since they can be replaced during evolution whilst the cell interactions and fates remain the same. Thus, insufficient consideration of phylogenetic and anatomical issues can lead to premature and overconclusive statements of structural homology, as illustrated by the recent debate about the origins of bilaterality. In addition, evo-devo researchers need to make an effort to depart from the “basal baloney” and “ancestral attractions” [102]. There has been an unfortunate tendency to use the “basal” label as a kind of magic time machine, leading to faulty chains of reasoning favouring conservation over divergence, such as: (i) the sea anemone is bilateral; (ii) the sea anemone is a basal cnidarian; (iii) the cnidarian are basal with respect to bilaterians; thus (iv) the ancestor of cnidarians and bilaterians was bilateral. Likewise, some of the supporters of the sea anemone model tend to disregard data from other cnidarian lineages (notably from the hydrozoans), considering the “basal” sea anemone more representative of an “ancestral” cnidarian. But Nematostella, being a member of Edwardsiidae, is a strongly modified sea anemone [43]. More importantly, anybody familiar with tree-thinking will understand that, within Cnidaria, the Medusozoa (to which hydrozoans belong) are exactly as basal as the anthozoans are (provided that both are monophyletic sister-groups), and similarly, that among Metazoa, the Bilateria are exactly as basal as the Cnidaria are. Moreover, “basal” lineages do not necessarily retain ancestral character states [14,35,102]. The anthozoans, for example, have many apomorphies (e.g. pharynx, siphonoglyphs, retractor muscles: precisely those structures that are affected by bilaterality in this group) and thereby for all of these characters they are more derived than the hydrozoans (under the hypothesis of anthozoan monophyly).
Common themes are emerging from studies on the molecular mechanisms involved in polarity and symmetry among eukaryote lineages that have acquired multicellularity independently from the metazoans. This includes the role of diffusible signals in pattern formation (for example in the fucus embryo [26] or in plant leaf polarity [106]) and in directing chemotactic cell movement (e.g. in the Dictyostelium multicellular slug [56]), the role of transcription factors in maintaining and refining spatial differences (well-studied in plants [106,186]), the importance of antagonism between regulatory pathways [106], and the high frequence of convergent changes in symmetry associated with strong adaptive value (e.g. recurrent evolution of floral zygomorphy in angiosperms [38,46]). These common characteristics represent analogous solutions to the same constraints, and reflect the inherent potential of the eukaryote cell for evolving more or less complex forms of multicellular organism geometry.
Acknowledgements
I am extremely grateful to Evelyn Houliston for in-depth comments and suggestions which greatly improved the manuscript, particularly concerning developmental and molecular aspects of polarity. Many thanks to Alexander Ereskovsky for providing original illustration and data on homoscleromorph radial anatomies and precious advice on the manuscript. I acknowledge Nicole Boury-Esnault and Jean Vacelet, the organisers of the Porifera Training Course held in Marseille in July 2005, and Bernard Picton and Thierry Perez for original pictures of demosponges. Alexandre Alié provided the Pleurobrachia picture. I thank Eric Quéinnec, Muriel Jager, Nicolas Rabet, Roxane Chiori, Lucas Leclère, Alexandre Alié, Nicole Boury-Esnault, Stéphane Schmitt and Hervé Le Guyader for insightful comments and discussion on the manuscript. This work was supported by GIS “Institut de la Génomique Marine” – ANR “programme blanc” NT_NV_52 Genocnidaire.