1 Introduction
The rice striped stem borer, Chilo suppressalis Walker (Lepidoptera: Pyralidae), is a destructive pest in rice fields around the world [1]. This pest was reported in Iran in 1973 and was widely distributed in all paddy fields [2]. Chemical pesticides, especially organophosphorus (Ops) compounds, have been a common control procedure, although other methods such as cultural practices (e.g., ploughing), biological control (by parasitoids and entomopathogenic fungi) and pheromone traps (by using Z-13, octadecenal, Z-11, hexadecenal and Z-9, hexadecenal) have been incorporated, recently [3].
α-glucosidase (EC 3.2.1.20) catalyzes the hydrolysis of terminal, non-reducing 1,4-linked alpha-D-glucose residues while releasing alpha-D-glucose. This enzyme strongly hydrolyzes sucrose, maltose, maltodextrin and pNP-α-D-glucopyranoside. It could be found in alimentary canal and salivary secretions of insects; in some insect such as Apis melifera L., it was found in the hypopharyngeal glands [4,5]. β-glucosidase is an enzyme which acts upon bonds among two glucose or glucose-substituted molecules and hydrolyzes these major bonds (; i.e., the disaccharide cellobiose). Beta-glucosidases are required by organisms (some fungi, bacteria, termites) which consume carbohydrates having linkages and play an important role in insect digestion [4]. These enzymes are active upon a large range of components in plants. These components are involved in plant resistance to insects and insect predation by herbivory. The majority of these components are L-glycosides that release cyanide (or other toxic compounds) upon hydrolysis and are toxic for many herbivorous insects [4].
Many insects, including the rice striped stem borer, which constitute serious pests of cereals (polysaccharide-rich diet) are dependent on their glycosidases (α-amylase, glucosidases and galactosidases) for survival. In insects, five digestive glucosidases including α-glucosidase (hydrolyzes maltose, sucrose, trehalose, melezitose, raffinose, stachyose), β-glucosidase (hydrolyzes cellobiose, gentibiose, methyl β-glycoside), α-galactosidase (hydrolyzes melibiose, raffinose, stachyose), β-galactosidase (lactose) and β-fructofuranosidase (sucrose and raffinose) are known to be exist [6,7]. α- and β-glucosidases have been isolated and characterized from many insects including Dysdercus peruvianus Herrich-Schaffer (Hemiptera: Pyrrhocoridase), Sitophilus zeamais L. (Coleoptera: Curculionidae), Apis mellifera L. (Hymenoptera: Apidae), Drosophila melanogaster L. (Diptera: Drosophillidae), Musca domestica L. (Diptera: Muscidae) and Thaumetopoea pityocampa Schiffermuller (Lepidoptera: Notodontidae) [7–10].
By considering the importance of carbohydrate digestion as a target for rice striped stem borer control, a study on their digestive enzymes could definitely be crucial in adopting of new control procedures. As a strategy of control, inhibitors of insect digestive enzymes have already been demonstrated to be an important biotechnology system in the control of insect pests especially by using resistant varieties [11–14]. In addition, glucosidases have an important role in plant-herbivorous co-evolution, because of their importance in break down of secondary metabolites of plants [15,16]. So, the aim of the present study was to isolate and characterize α- and β-glucosidases of C. suppressalis to gain a better understanding of the digestive physiology of the insect. This understanding will hopefully lead to new and winning management strategies for control of this pest.
2 Materials and methods
2.1 Insect
Insects were reared by using a method described by Kammano and Sato [17] (, light cycle 16L: 8D and RH > 80%). To decrease the negative effects of laboratory mass culture, 400 pupae were collected from fields by dissecting the rice stems and reared on the same variety of seedling (Taroum) as the sampling sites. Hatched larvae were fed on rice seedlings until larvae reached to the 4th stadium, 30 larvae in three replicates were randomly selected for biochemical analysis. Stadia were determined using Dayer's formula as previously described [18].
2.2 Sample preparation and enzyme assays
Midguts and salivary glands of 4th instar larvae were removed by dissection under a stereo microscope in ice-cold saline buffer (6 μmol/L NaCl). Tissues were rinsed in ice-cold buffer, placed in a precooled homogenizer and ground in 1 mL of universal buffer (0.02 M) containing succinate, glycine and 2-morpholinoethanesulfonic acid (pH 6.5) [19]. Homogenates were transferred to 1.5 mL centrifuge tubes and centrifuged at for 20 min at 4 °C. The supernatants from each tissue were pooled then stored at −20 °C for subsequent analyses.
For solubilization of membrane hydrolyses (α-, β-glocusidases) in Triton X-100, membrane preparations were exposed to Triton X-100 for 20 h at 40 °C, in a ratio of 10 mg of Triton X-100/mg of protein, before being centrifuged at for 30 min. No sediment was visible after the centrifugation of this supernatant at for 60 min. The activity of the enzymes remains unchanged, at −20 °C, for periods of at least a month [20].
α-, β-glocusidases activities were assayed by incubating 50 μL of enzyme solution with 75 μL of p-nitrophenyl-α-D-glucopyranoside () (5 mM), p-nitrophenyl-β-D-glucopyranoside () (5 mM) and 125 μL of 100 mM universal buffer (pH 5.0) at 37 °C for 10 min. The reaction was stopped by adding 2 mL of sodium carbonate (1 M) [20].
Parry's procedure [21] was conducted to find standard curve of glucosidases. Hence, series of p-nitrophenyl concentrations (0.0625–1 mg/mL) were provided and 10 μl of each concentration were added to buffer to reach final 0.625, 1.25, 2.5, 5, 10 mmol concentrations. In three replicates, 1 ml of NaOH solution (0.25 M) added to each well after 60 min and absorbance was read at 405 nm.
2.3 Effect of pH and temperature on enzyme activity
The effect of temperature and pH on α- and β-glocusidases activities were examined using these enzymes' extractions from the midgut and salivary glands of larvae. The effect of temperature on α- and β-glocusidases activities were determined by incubating the reaction mixture at 20, 25, 30, 35, 37, 40, 45, 50, 55, 60 and 70 °C for 30 min, followed by measurement of activity. Optimal pH for their activities was determined using universal buffer with pH set at 3, 4, 5, 6, 7, 8, 9, 10, 11 and 12.
2.4 Effect of activators and inhibitors on enzyme activity
To test the effect of different ions (which are presents in many plants and feeding compounds) on the enzymes, midguts and salivary glands of 4th instar larvae were dissected in distilled water. Enzyme assays were performed in the presence of different concentrations of chloride salts of Na+ (5, 10, 20 and 40 mmol/L), K+ (5, 10, 20 and 40 mmol/L), Ca2+ (5, 10, 20 and 40 mmol/L), Mg2+ (5, 10, 20 and 40 mmol/L), and ethylenediaminetetraacetic acid (EDTA; 0.5, 1, 2 and 4 mmol/L), sodium dodecylsulfate (SDS; 1, 2 and 4 mmol/L) and Urea (0.5, 1, 2, 4, 6 and 8 mol/L). These compounds were added to the assay mixture, and activity was measured after 30 min incubation. A control was also measured (no compounds added) to calculate final relative activity.
2.5 Kinetic parameters ( and ) of α- and β-glucosidase in midgut and salivary glands
For the determination of enzyme kinetic parameters, twenty μl of appropriately diluted enzyme preparation were used in each assay. Final concentrations of substrate were 0.25, 0.5, 1, 1.5, 2, 2.5, 3 and 5 mM for both enzymes. The Michaelis constant () and the maximum velocity () were estimated by Sigmaplot software version 11 and the results of and were the of three replicates () for every tissue.
2.6 Protein determination
Protein concentration was measured according to the method of Bradford [22] using bovine serum albumin (Bio-Rad, München, Germany) as a standard.
2.7 Statistical analysis
Data were compared by one-way analysis of variance (ANOVA) followed by Tukey's student test when significant differences were found at [23].
3 Results
3.1 α- and β-glucosidase activity
α- and β-glucosidase activities were observed in the midgut and salivary glands of larval Chilo suppressalis larvae (Fig. 1). The activity of α-glucosidase was 0.009 and 0.0063 μmol/min/mg protein in midgut and salivary gland, respectively. The enzyme activity in midgut and salivary gland was 0.005 and 0.003 μmol/min/mg protein for β-glucosidase. There was a significant difference in measured glucosidases activity between midgut and salivary gland (Fig. 1). Overall data showed that activity of α-glucosidase in midgut and salivary glands of rice striped stem borer larvae is higher than that of β-glucosidase. Also, the standard calibration curve of p-nitrophenol is shown in Fig. 2.
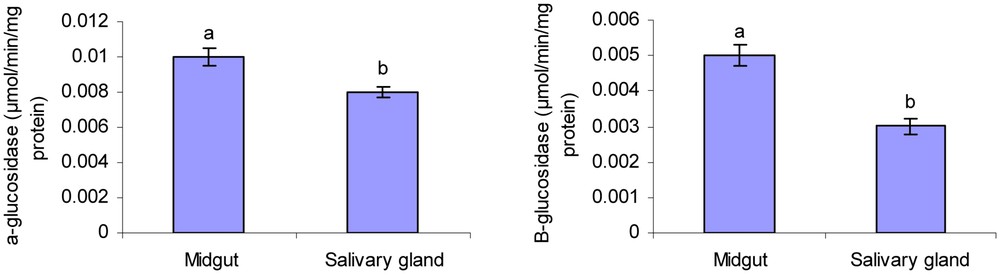
Activity level of α- and β-glucosidase in 4th instar larvae midgut and salivary glands of rice striped stem borer larvae. Different letters indicate that the activity of enzymes in different tissues are significantly different from each other by Tukey's test (p < 0.05).
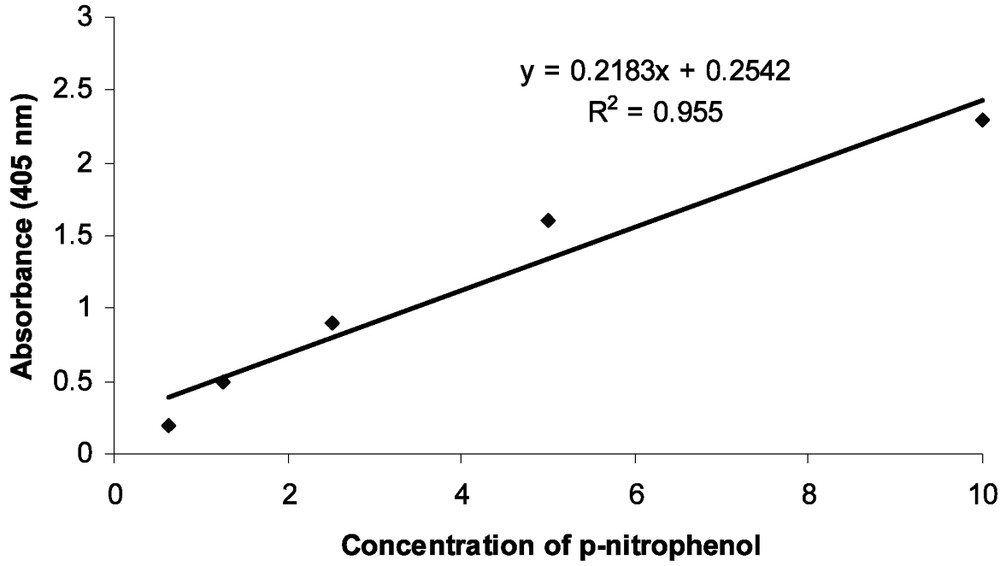
Standard calibration curve for the determination of p-nitrophenol released in the α- and β-glucosidases assay (y = 0.2183x + 0.2542; ).
3.2 Effect of pH and temperature on enzyme activity
The α- and β-glucosidase activity in midgut and salivary glands of larvae increased steadily from pH 3 to 8, and then decreased with increasing pH and there were significant differences among measured values for each pH (Table 1). α- and β-glucosidase were considerably active over a broad range of temperatures. As the results show, the optimal temperature for α- and β-glucosidase activities was 45 °C for both midgut and salivary gland (Table 2).
Effect of pH on activity of α- and β-glocusidases extracted from midgut and salivary gland of rice striped stem borer.
pH | α-glucosidase | β-glucosidase | ||
Midgut | Salivary glands | Midgut | Salivary glands | |
3 | 0.034 ± 0.01 d | 0.04 ± 0.001d | 0.023 ± 0.003 d | 0.019 ± 0.001 d |
4 | 0.042 ± 0.001 c | 0.041 ± 0.001 d | 0.034 ± 0.001 c | 0.03 ± 0.005 c |
5 | 0.053 ± 0.001 bc | 0.055 ± 0.0007 bc | 0.046 ± 0.002 b | 0.037 ± 0.006 c |
6 | 0.063 ± 0.0006 b | 0.069 ± 0.002 ab | 0.053 ± 0.002 b | 0.042 ± 0.002 b |
7 | 0.072 ± 001 ab | 0.073 ± 0.002 a | 0.068 ± 0.008 a | 0.05 ± 0.003 ab |
8 | 0.083 ± 002 a | 0.078 ± 0.001 a | 0.071 ± 0.004 a | 0.058 ± 0.007 a |
9 | 0.072 ± 0.003 ab | 0.075 ± 0.001 a | 0.062 ± 0.005 ab | 0.055 ± 0.003 a |
10 | 0.072 ± 0.001 ab | 0.067 ± 0.003 ab | 0.06 ± 0.003 ab | 0.049 ± 0.003 ab |
11 | 0.063 ± 0.001 b | 0.06 ± 0.0007 b | 0.048 ± 0.008 b | 0.04 ± 0.001 b |
12 | 0.042 ± 0.003 c | 0.051 ± 0.0009 c | 0.031 ± 0.007 c | 0.033 ± 0.004 c |
Effect of temperature on activity of α- and β-glocusidases extracted from midgut and salivary gland of rice striped stem borer.
Temperature | α-glucosidase | β-glucosidase | ||
Midgut | Salivary glands | Midgut | Salivary glands | |
20 | 0.047 ± 0.004 c | 0.018 ± 0.0003 c | 0.037 ± 0.002 f | 0.029 ± 0.003 cd |
30 | 0.054 ± 0.005 bc | 0.032 ± 0.004 b | 0.044 ± 0.005 e | 0.037 ± 0.009 c |
35 | 0.062 ± 0.007 b | 0.044 ± 0.003 ab | 0.052 ± 0.003 d | 0.049 ± 0.007 b |
40 | 0.096 ± 0.005 a | 0.052 ± 0.006 a | 0.086 ± 0.007 a | 0.064 ± 0.001 a |
45 | 0.055 ± 0.01 bc | 0.043 ± 0.0004 ab | 0.075 ± 0.02 b | 0.06 ± 0.003 a |
50 | 0.066 ± 0.008 b | 0.034 ± 0.003 b | 0.066 ± 0.002 c | 0.043 ± 0.001 b |
55 | 0.05 ± 0.005 c | 0.028 ± 0.0006 bc | 0.047 ± 0.004 e | 0.034 ± 0.002 c |
60 | 0.037 ± 0.001 cd | 0.021 ± 0.002 c | 0.031 ± 0.001 f | 0.028 ± 0.001 cd |
70 | 0.023 ± 0.002 e | 0.016 ± 0.0008 d | 0.019 ± 0.003 g | 0.02 ± 0.05 d |
3.3 Effect of activators and inhibitors on enzyme activity
Severally used chemical compounds had different effects on activity levels of α- and β-glucosidase in midgut and salivary glands of rice striped stem borer larvae, although they had a similar effect on both enzymes (Tables 3 and 4). Activity level of α- and β-glucosidase in midgut and salivary glands of rice striped stem borer elevated due to increasing of NaCl, CaCl2 and MgCl2 concentrations (Tables 3 and 4). Activity level of enzyme decreased due to using KCl, EDTA, SDS and Urea both in midgut and salivary glands. There were significant differences in α- and β-glucosidase activity due to using different compounds in midgut and salivary gland of rice striped stem borer larvae in comparison with control (Tables 3 and 4).
Relative activity of Chilo suppressalis α-glucosidase toward different compounds.
Compounds | Concentration (mmol/L) | Relative activity (midgut) | Relative activity (salivary gland) |
Control | – | 100 | 100 |
NaCl | 5 | 112 | 96.58 |
10 | 172.32⁎ | 134.67⁎ | |
20 | 174.41⁎ | 156.6⁎ | |
40 | 223.25⁎ | 193.54⁎ | |
CaCl 2 | 5 | 139.08⁎ | 124.58⁎ |
10 | 153.69⁎ | 152.03⁎ | |
20 | 187.93⁎ | 168.75⁎ | |
40 | 225.53⁎ | 190.24⁎ | |
KCl | 5 | 50⁎ | 83.01 |
10 | 27.9⁎ | 81.13 | |
20 | 30.62⁎ | 45.28⁎ | |
40 | 36.04⁎ | 29.26⁎ | |
MgCl 2 | 5 | 143.73⁎ | 116.74 |
10 | 179.82⁎ | 133.09⁎ | |
20 | 201.34⁎ | 149.3⁎ | |
40 | 206.2⁎ | 166⁎ | |
EDTA | 0.5 | 91.93 | 101 |
1 | 86.04 | 86.37 | |
2 | 63.27⁎ | 70⁎ | |
4 | 55⁎ | 63.01⁎ | |
SDS | 2 | 100.62 | 103.28 |
4 | 93.72 | 99.15 | |
6 | 101.04 | 78.16 | |
8 | 86.72 | 60.41⁎ | |
Urea | 1000 | 58.58⁎ | 86.79 |
2000 | 63.39⁎ | 60.83⁎ | |
4000 | 46.51⁎ | 52.37⁎ | |
5000 | 40.76⁎ | 44.01⁎ | |
6000 | 26.74⁎ | 38.26⁎ |
⁎ control.
Relative activity of Chilo suppressalis β-glucosidase toward different compounds.
Compounds | Concentration (mmol/L) | Relative activity (midgut) | Relative activity (salivary gland) |
Control | – | ||
NaCl | 5 | 99.95 | 89.89 |
10 | 111.11 | 112.12 | |
20 | 171.79⁎ | 144.44⁎ | |
40 | 198.57⁎ | 163.63⁎ | |
CaCl 2 | 5 | 120.21⁎ | 104.73 |
10 | 158.47⁎ | 150.42⁎ | |
20 | 192.91⁎ | 178.11⁎ | |
40 | 233.33⁎ | 193.31⁎ | |
KCl | 5 | 108.54 | 80.7⁎ |
10 | 69.23⁎ | 78.78⁎ | |
20 | 65.29⁎ | 64.2⁎ | |
40 | 58.03 | 45.95⁎ | |
MgCl 2 | 5 | 100.45 | 102.9 |
10 | 180.41⁎ | 147⁎ | |
20 | 186.32⁎ | 166.6⁎ | |
40 | 200⁎ | 169.49⁎ | |
EDTA | 0.5 | 110.82 | 83.83 |
1 | 67.35⁎ | 62.42⁎ | |
2 | 64.10⁎ | 72.72⁎ | |
4 | 46.49⁎ | 29.8⁎ | |
SDS | 2 | 97.93 | 79.66⁎ |
4 | 77.77⁎ | 55.55⁎ | |
6 | 68.88⁎ | 59.59⁎ | |
8 | 61.32⁎ | 43.75⁎ | |
Urea | 1000 | 74.7⁎ | 70.46⁎ |
2000 | 70.9⁎ | 48.4⁎ | |
4000 | 53.8 | 44.94 | |
5000 | 58.11⁎ | 21.2⁎ | |
6000 | 56.41⁎ | 20.05 |
⁎ control.
3.4 Kinetic parameters ( and ) of α- and β-glucosidase im midgut and salivary glands
Kinetic parameters of glucosidases are shown in Table 5 and Fig. 3. As can be seen in Table 5, alpha-glucosidase of midgut and salivary glands were 0.07 and 0.057 (μmol/min/mg protein) and alpha-glucosidase values were 1.71 and 1.24 (mM), respectively. Beta-glucosidase value were 0.031 and 0.61 (μmol/min/mg protein) and values were 0.61 and 0.39 (mM) in midgut and salivary glands (Table 5 and Fig. 3).
Kinetic parameters ( and ) of α- and β-glucosidase in rice striped stem borer larvae.
Tissues | α-glucosidase | β-glucosidase | ||
(μmol/min/mg protein) | (mM) | (μmol/min/mg protein) | (mM) | |
Midgut | 0.07 ± 0.0048 a | 1.71 ± 0.048 a | 0.031 ± 0.0033 a | 0.61 ± 0.1 a |
Salivary glands | 0.057 ± 0.0034 b | 1.24 ± 0.059 b | 0.02 ± 0.009 a | 0.39 ± 0.0093 b |
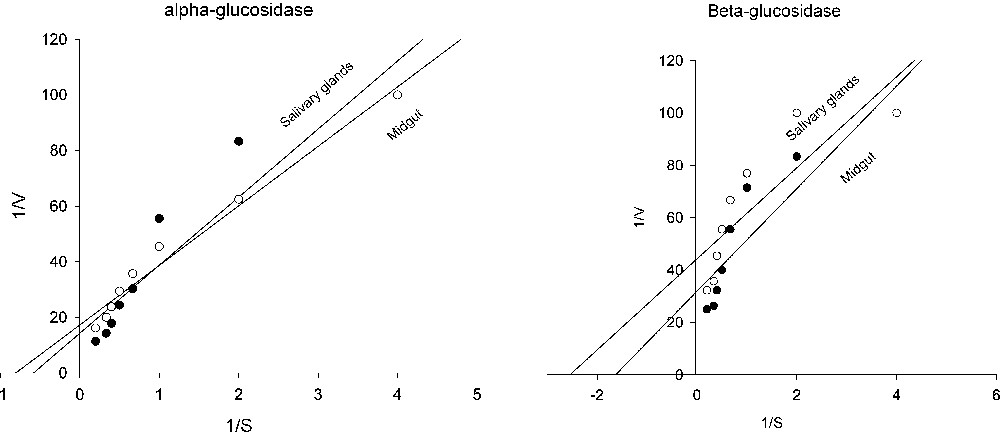
Lineweaver–Burk plots of alpha and beta-glucosidase in salivary glands and midgut of rice striped stem borer larvae.
4 Discussion
The present study clearly depicted that the larvae of Chilo suppressalis have α- and β-glucosidase activities both in the midgut and salivary glands. Rice striped stem borer can digest polysaccharides partially by salivary secretions which would be ingested along digesting of starches to maltose, and then hydrolyzed to glucose by α- and β-glucosidase. Complete breakdown of carbohydrates should take place in the midgut where large amounts of amylase, α- and β-glucosidase exist [24,25].
Activity level of α- and β-glucosidase in midgut is higher than salivary gland of rice striped stem borer which these results agrees with previous reports. Horie [26] showed that glucosidases activity was low in Coreidae and Pentatomidae (Hemiptera) and in some species there was no β-glucosidase activity at all. Terra and Ferreira [27] demonstrated that α-glucosidase hydrolyzes di- and oligosaccharides which are known as maltase or sucrase. These enzymes are found in alimentary canal of almost all insects and type of food causes their selective effectiveness on substrate. For example, Marinotti and James [28] showed that α-glucosidase converts oligosaccharides to maltopentose in Aedes aegypti L. (Diptera: Culicidae). In honey bees, because of presence of more sucrose than maltose, α-glucosidase hydrolyzes sucrose better than maltose but in lepidopteran larvae it is vice versa. Esen [29] represented that β-glucosidases were found in all plants, fungi, bacteria and animals especially major orders of insects. This enzyme is present in insects that feed on cellulose and has an important role in hydrolyzing hemicellulotic components. These enzymes are active upon a large range of substrates and in some cases they are involved in plant resistance to insect feeding, because of the occurrence of L-glycosides in many plants that release cyanide (or other toxic compounds) upon hydrolysis [4].
Results from this study showed that α- and β-glucosidase activities of Chilo suppressalis have activity in a broad pH range between 3 to 8 (i.e. increasing rate) and the suitable pH was observed at 8 in both midgut and salivary glands. In other lepidopteran larvae, the optimal pH for disaccharidases and specific glycosidases ranges between 5.3 for trehalase in wax moth Galleria mellonella L. (Diptera: Pyralidae) [30] and 8.0 for α-glucosidase in 3rd instar larvae of E. vitella [31]. Alkaline pH optima for the assayed glucosidase in Parnassius apollo ssp. frankenbergeri Stoll (Lepidoptera: Papilionidae) larvae were similar to the ones stated for the majority of the examined lepidopterans [32]. These differences may reflect the phylogenetic relationship [7] or response to different food sources. Also there may be a difference attributable to the origin of the enzyme, i.e. gut or salivary glands. These data show that there is a correlation between optimal pH of glucosidases and luminal pH in insect midgut [7]. It has been suggested that high gut pH is a type of adaptation for such insects to feed on plant materials which enrich with tannins [33]. Tannins bind to proteins at lower pH values then reducing the efficiency of digestion [34].
In this study, α- and β-glucosidases of rice striped stem borer have an optimal activity in 45 °C for both midgut and salivary glands, respectively and activity increased steadily from 20–45 °C. These results coincide with Huber and Mathison studies [9] in which they demonstrated that optimal activity of glucosidases was taken place at 20–45 °C. Takenaka and Echigo [35] also showed that optimal temperature for glucosidase activity is between 20–50 °C. Although, biological reactions are catalyzed by proteinaceous enzymes, and each enzyme has a temperature which its three-dimensional structure is disrupted by heat. Therefore, biological reactions occur faster with increasing temperature up to the point of enzyme denaturizing, above which above of it enzyme activity and the rate of the reaction decreases sharply [36–38].
Data showed that NaCl, MgCl2 and CaCl2 increased the activity level of glucosidases in rice striped stem borer. Zeng and Cohen [38] showed that Cu2+ had the most effect (118%) and Mg2+ the least effect (96%) on α- and β-glucosidase of Lygus Hesperus Fabricius (Hemiptera: Miridae). However, it has been reported that glucosidases are metalloproteins that require calcium for maximum activity. Calcium also affords stability for the enzymes from variety of sources (including insects) to both pH and temperature extremes [8]. The other features of this enzyme, such as sensitivities to chelating agent (EDTA), Urea and SDS, are typical to many animal glucosidases [4].
Analysis of Lineweaver-Burk plots (Table 5 and Fig. 3) provide information regarding glucosidase activities in rice striped stem borer larvae. By considering the kinetic assays, important differences were also observed according to the used substrate. Since the has an inverse relationship with the substrate concentration required to saturate the active sites of the enzyme, increasing of value indicates decreased enzyme affinity for substrate. In the other words, is the measurement of the stability of the enzyme-substrate complex and a high would indicate weak binding and a low strong binding [2].
The physiological role of the glucosidases is to release glucose from dietary small oligosaccharides which then will be absorbed by midgut epithelim. Glycosidases are exoenzymes which hydrolyse the o-glycosyl bonds of carbohydrates [4]. Almost all naturally occurring plant glycosides are β-linked o-glycosyl compounds hence, β-glycosidases are the key enzymes in the metabolism of plant glycosides. Plants produce a wide variety of allelochemicals which act as defensive compounds. These include alkaloids, cyanogenic and triterpenoid glycosides, phenols, flavenoids and nonprotein amino acids [39]. Among these allomones, glycosides seem to play an important role in host plant resistance to insects. For example, tomatine, an alkaloid glycosides and rutin (quercetin 3-rutinoside) are involved in the resistance of tomato to the tomato fruitworm [Heliothis zea Fabricius (Lepidoptera: Noctuidae)] by acting as feeding deterrents [10]. Tomatine was also related to the resistance of tomato to the Colarado potato beetle [Leptinotarsa decemlineata Say (Coleoptera: Chrysomelidae)] [39]. DIMBOA is another glycoside which is present in young corn tissues. DIMBOA is involved in resistance of corn to the European corn borer [Ostrinia nubilalis Hubner: Lepidoptera: Pyralidae)]. In addition numerous plant glycosides have been found to possess antifeedant activity toward phytophagous insects. For example, the phenolic glucoside phloridzin was found to be a deterrent to non-apple feeding aphids, Myzus persicae Sulzer (Hemiptera: Aphididae) and Amphorophora agathomica Passerini (Hemiptera: Aphididae) and the alkaloid glucosides solanine and chaconine inhibited feeding of Colorado potato beetle. Sinigrin, a mustard oil glucoside (glucosinolate), was found to act as a chemical barrier to Papilio polysenes Stoll (Lepidoptera: Papilionidae) by reducing larval survival and the number of fertile eggs laid by surviving adults. The toxic action of these glycosides is due to their corresponding aglycones liberated by the action of β-glucosidase. Therefore, high β-glucosidase activity is detrimental to phytophagous insects when they ingest a plant glycoside [4]. Decreased levels of digestive enzymes at higher concentration of some compounds in plant extract suggest reduced phosphorous liberation for energy metabolism, and decreased rate of metabolism, decreased rate of transport of metabolites and may be due to the direct effects of plant extract on enzyme regulation [40,41]. These compounds interfere with growth via digestive impairment by inhibiting the secretion of trypsin-type proteinases from gut epithelial cells and disrupt the digestion and/or absorption of ingested food and with the conversion of absorbed food to biomass [42,43].
Study of β-glucosidase in herbivorous insects is so important not only for understanding of digestion biochemistry but also for developing of insect pest management strategies. If the specific substrate of β-glucosidase is identified, it could be used to develop of resistance varieties. Genetic engineering techniques will be able to transfer genes producing toxic compounds to target plants so that it causes plant resistance against herbivorous insect. Controlling of pests with usage of resistant varieties is one of the most important practices that are dependent on inhibitors in plants. Hence, characterization of digestive enzymes especially examination of inhibition effects on enzyme activity could be useful. Successful results have in the past been obtained by inhibitors that completely inhibited their target enzymes but recent results show that even partial inhibition can give substantial control of insect pests [44]. Nevertheless, the use of nonproteinacious inhibitors for production of insect-resistant transgenic plants is much more difficult. Hence, the presence of multiple expressed transgenes would be required in order to confer protection [5]. Thus, it is possible to utilize this knowledge to develop plant varieties with more plant defensive system. This could be achieved through traditional breeding programs to select plants possessing higher degrees of resistance factor i.e. toxic glycosides that are not easily degraded.
Acknowledgement
This research was supported by Rice Research Institute of Iran (IRRI) and a University of Tehran grant. We sincerely appreciate M. Belbasi and Z. Hashemi for their assistance and thank two anonymous reviewers for their useful comments.