1 Introduction
Increase in environmental pollution caused by heavy metals, due to industrial and agricultural activities, is becoming a significant problem in the world [1]. Copper, an essential micronutrient, plays a vital role in maintaining normal metabolism in higher plants. It is involved in a wide range of biochemical and physiological processes. It also participates in electron-transfer reactions of photosynthesis in the form of plastocyanin [2]. Copper at high levels becomes strongly phytotoxic to cells causing inhibition of plant growth or even death [3]. Studies from some plant species demonstrate that excess Cu in plant growth medium induces formation of reactive oxygen species in treated tissues [4]. Cu-induced generation of hydrogen peroxide, hydroxyl radicals, or other reactive oxygen species (ROS) has been directly correlated with the damage to proteins and lipids [5]. Photosynthesis is also sensitive to excessive Cu, and the pigment and protein components of photosynthetic membranes are the targets [6]. In addition, Cu toxicity is related to disturbances in the uptake of other essential elements [6]. Enzymatic scavengers, such as superoxide dismutase, are involved in the detoxification of , peroxidase, catalase, and enzymes of the ascorbate glutathione cycle are related to the removal of H2O2 [5]; and non-enzymatic radicals scavengers such as ascorbate and glutathione have been interpreted as the key antioxidants for the removal of H2O2 in plant cells, thus reducing the accumulation of the free radicals [7]. Evidence from several plant species reveals that Cu caused oxidative stress by mediating the activities of antioxidative enzymes [7,8]. The mechanisms by which Cu induces antioxidative responses, whether different plant species share a common defense mechanism or not, are not yet fully understood. The inhibition of plant growth and crop production by excess heavy metals such as copper, cadmium, zinc, or nickel in contaminated soil is a global agricultural problem. The excessive metals from soil and aqueous streams can be removed using metal-accumulating plants has been proposed as a solution [9]. However, in addition to the knowledge of uptake, translocation, or compartmentation of heavy metals in plants, understanding of the tolerance mechanisms to improve plants of biotechnological interest is also important [10]. Among metabolic changes, the antioxidative defense mechanisms of plants were of particular interest. We were therefore interested to test the hypothesis that Cu excess in a growth medium induces oxidative stress and ultrastructural alterations with Cu concentration dependent variability and activates defense system in freshly grown water lettuce. Induction of oxidative stress was investigated in terms of , H2O2 content in roots and leaves of this plant and activation of defense system in terms of SOD (E.C.1.15.1.1), POX (E.C.1.11.1.7) and CAT (E.C.1.11.1.6) activity. We also hypothesize that there is a relationship between a level of individual ROS and activity of some antioxidant enzymes. Copper, though an essential micronutrient for plants, it can also become toxic when applied in amounts higher than its optimal level, particularly catalyzing the formation of harmful free radicals. The simultaneousness of enzymatic responses suggested that induction of these various enzymes is part of a general defense strategy to cope with overproduction of reactive oxygen species.
2 Results and discussion
2.1 Effects of Cu on growth and accumulation
A significant result of the present investigation is the change in the dry biomass of roots and leaves under short-term copper exposure. It has been proposed that low levels of ROS serve as internal signals and are an important factor for inducing mitosis related growth processes [11]. The inhibition of growth was seen from the initial hours of Cu exposure. Plants exposed to 100 μM Cu showed significant decrease in growth of the plants (Fig. 1). Copper accumulation in both roots and leaves showed a dose dependent increase (Fig. 2). The increase in the Cu accumulation was higher in leaves as compared to roots. Pistia being accumulator showed significant increase after 24 h as compared to the control plants. Copper is an essential micronutrient, readily taken up by the plants through membrane transporters [12,13]. The higher accumulation of Cu in Pistia plants may result in the activation of a putative Cu transporter and also may result in the distortion of plasma membrane.
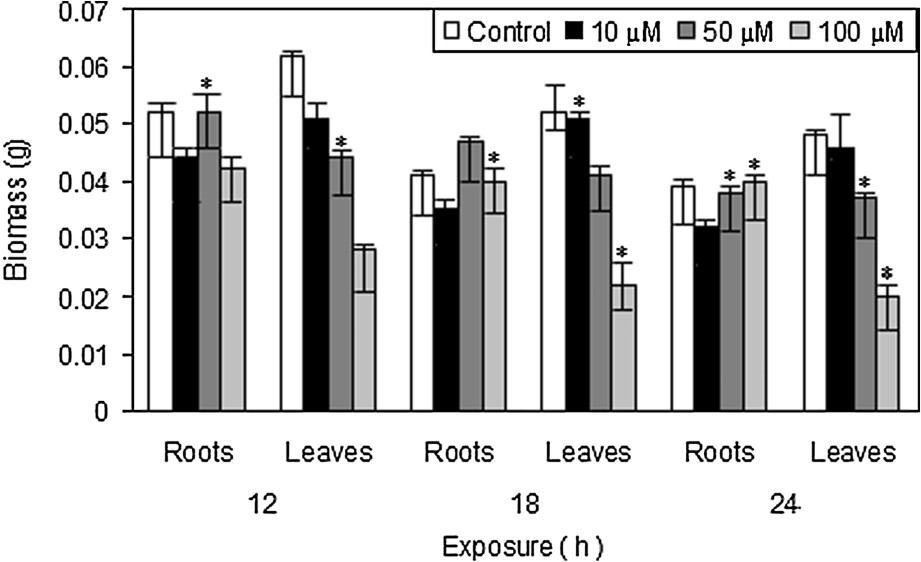
Effect of CuSO4 on root and leaf dry matter production in Pistia stratiotes L. after 12, 18 and 24 h of exposure. The data presented are means of three replicates. ∗ represents the significance level at P ⩽ 0.05 when compared with control.
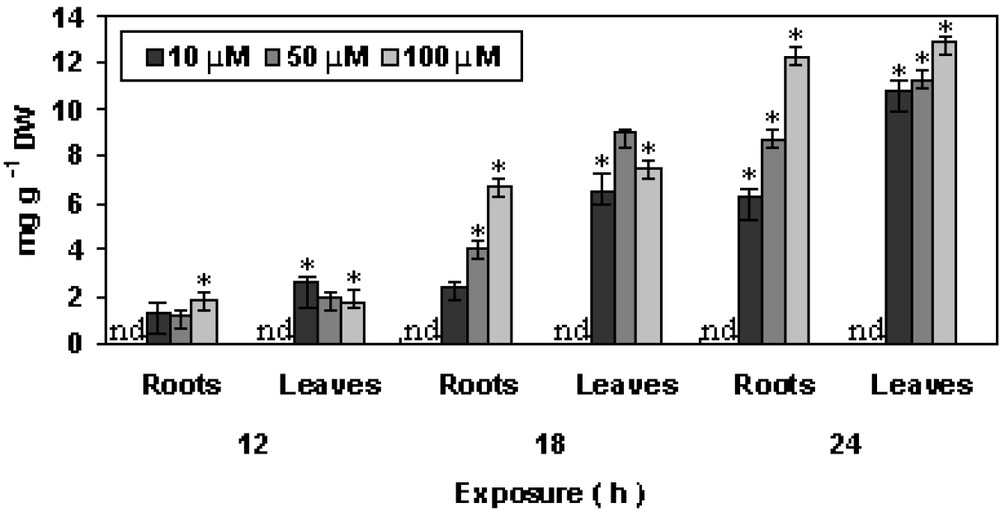
Changes in accumulation of Cu in P. stratiotes L. after 12, 18 and 24 h of exposure. The data presented are means of three replicates. ∗ represents the significance level at P ⩽ 0.05 when compared with control.
No Cu accumulation could be detected in case of control plants.
2.2 Effects of Cu on photosynthetic pigments
The degradation of chlorophyll pigments may eventually decrease photosynthetic efficiency in plants which might be one of the potent causes of reduction in growth of plant [14,15]. Both chlorophyll and carotenoid contents showed almost similar response upon copper exposure (Tables 1 and 2). Initially increased but then decreased down gradually with time of exposure. A similar finding has also been reported in other plants [16–18]. The declaim in chlorophyll and carotenoid contents can be regarded as a metal specific response that may have resulted in chlorophyll degradation and inhibition of photosynthesis [19]. The carotenoid content showed increased initially at 50 and 100 μM Cu on 18 h followed by decreased at higher metal concentrations. At 24 h, carotenoid content decreased sharply to 51.2% as compared to respective control.
Effect of CuSO4 on chlorophyll contents (μmol g−1 dw) in Pistia stratiotes L. at different concentrations and exposure.
Con. (μM) | Exposure (h) | ||
12 | 18 | 24 | |
Control | 0.918 ± 0.03 | 0.882 ± 0.02 | 0.088 ± 0.02 |
10 | 0.902 ± 0.02⁎ | 0.723 ± 0.03 | 0.712 ± 0.02⁎ |
50 | 0.881 ± 0.02⁎ | 0.702 ± 0.04⁎ | 0.681 ± 0.04⁎ |
100 | 0.808 ± 0.03⁎ | 0.681 ± 0.02⁎ | 0.612 ± 0.02⁎ |
⁎ represents the significance level at when compared with control.
Effect of CuSO4 on carotenoid contents (μmol g−1 dw) in Pistia stratiotes L. at different concentrations and exposure.
Con. (μM) | Exposure (h) | ||
12 | 18 | 24 | |
Control | 0.321 ± 0.02 | 0.332 ± 0.05 | 0.231 ± 0.05 |
10 | 0.311 ± 0.03⁎ | 0.304 ± 0.04⁎ | 0.181 ± 0.21⁎ |
50 | 0.280 ± 0.02 | 0.248 ± 0.02 | 0.181 ± 0.21⁎ |
100 | 0.128 ± 0.02⁎ | 0.234 ± 0.05⁎ | 0.182 ± 0.03⁎ |
⁎ represents the significance level at when compared with control.
2.3 Effect of Cu on malondialdehyde (MDA) content
MDA is the decomposition product of polysaturated fatty acids (PUFA) of membranes and its increase shows plants are under high-level antioxidant stress [20]. As shown in Fig. 3, MDA content showed increased in activity, measured to analyze lipid peroxidation to assess the membrane damage imposed by copper. MDA increased in time-concentration dependent manner. The maximum increase in MDA content was observed in both roots and in leaves on 24 h at 100 μM, as compared to control. As reported earlier, heavy metal exposure promotes lipid peroxidation via free radical generation [18,21]. In the experiments, increase in MDA content prompted lipid peroxidation in Pistia plants, which indicated lipid peroxidation via free radical generation caused by enhanced production of H2O2 and in Pistia plants. This result is in accordance with the findings in Ceratophyllum dermersum L. [20,22].
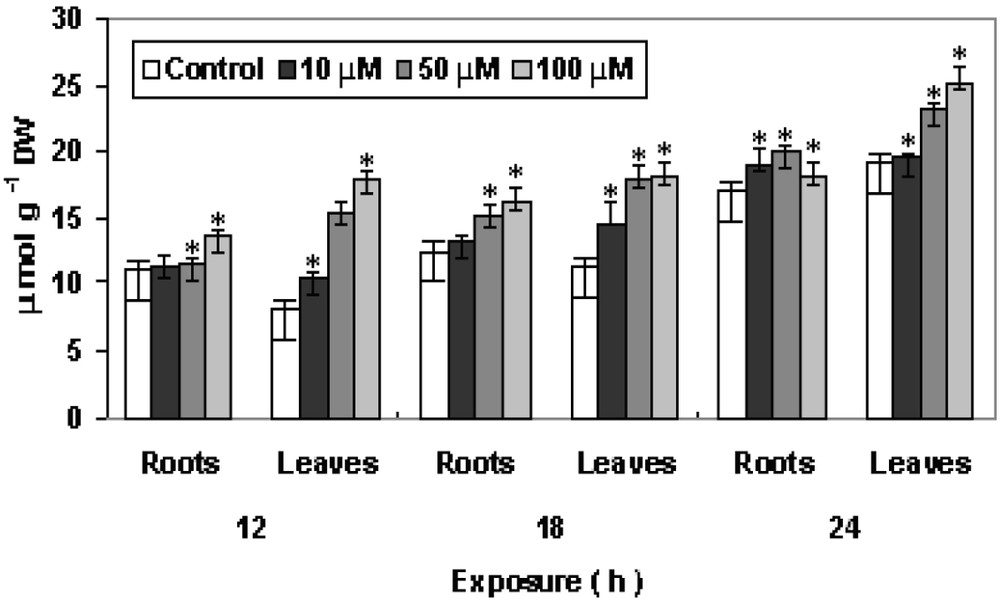
Changes in MDA contents in P. stratiotes L. after 12, 18 and 24 h of CuSO4 exposure. The data presented are means of three replicates. ∗ represents the significance level at P ⩽ 0.05 when compared with control.
2.4 Effects of Cu on hydrogen peroxide and superoxide radicals
Under stressful condition, toxic ROS are produced in cells. Pistia plants treated with Cu resulted in the generation of ROS like H2O2 and . H2O2 acts as a secondary messenger, ability to trigger various secondary responses in plants [23,24]. In the experiment, Cu resulted in increased production of H2O2, followed by increasing trends with increase in duration of exposure. Similar findings in H2O2 production has been reported for Cr, Pb and other heavy metals in case of moss and higher plants [17,18]. Copper promotes the generation of reactive oxygen species (ROS), H2O2 and . The changes in total peroxide and superoxide radical are shown in Fig. 4, A and B. Treatment of Cu after 24 h increased the H2O2 content with maximum increase at 100 μM in leaves as compared to control. After 24 h, the content showed significant increased in both roots and shoot as compared to control at 50 μM and 100 μM, respectively. The increase in concentration and duration of exposure resulted in high production. is moderately reactive and unable to cross the biological membrane, and together with H2O2 it forms toxic OH− that initiate lipid peroxidation resulting in distortion of biological membranes [17,25].

Changes in contents of (A) hydrogen peroxide and (B) superoxide radical in P. stratiotes L. after 12, 18 and 24 h of CuSO4 exposure. The data presented are means of three replicates. ∗ represents the significance level at P ⩽ 0.05 when compared with control.
2.5 Response of antioxidants to Cu toxicity
The antioxidant enzymes viz., POX, SOD and CAT showed varying responses at various concentrations and exposures (Fig. 5, A–C). The activity of POX remains increases slightly with increase in concentration and hour of exposure as compared to control. POX belongs to enzymes that has role in growth, development and senescence processes in plants. POX does not show initial increase. However, at 10 μM Cu has proved to be stimulatory during initial periods of treatment as reported earlier for other metals [15]. Apart from toxic H2O2 scavenging via POX activity in plants, it may also involve in the biosynthesis of cell wall component and lignification. The activity of SOD showed minor increase after 18 h in both roots and leaves, while after 24 h of treatment, activity was increased maximum in leaves and in roots at 100 μM as compared to control. SOD is an essential component of antioxidative defense system in plants. The induction of SOD by copper may indicate an acclimation response of the plants. SOD catalyzes the dismutation of to H2O2 and oxygen and is regarded as a first line of defense against ROS. Increase in SOD activity is related to oxidative stress tolerance [26]. CAT activity, on the other hand increase initially at 100 μM in roots on the 12 h treatment and gradually decreased with maximum at 100 μM in leaves on the 24 h treatment as compared to control. CAT scavenges the active oxygen species in plant cells. It participates in the main defense system against accumulation and toxicity of hydrogen peroxide and can play the role in controlling H2O2 level in cells. CAT showed initial increase after 12 h of Cu exposure but gradually decreased after 18 h. Similar observations were also reported in case of other plants [13,21,27].
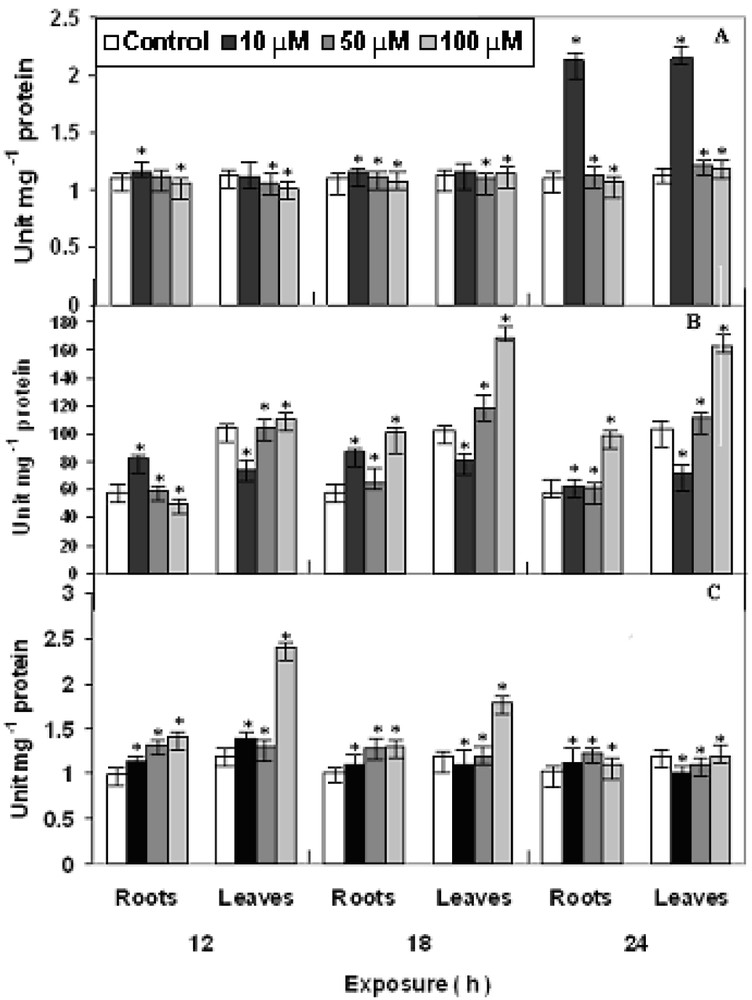
Changes in the activities of (A) POX, (B) SOD and (C) CAT in P. stratiotes L. after 12, 18 and 24 h of CuSO4 exposure. The data presented are means of three replicates. ∗ represents the significance level at P ⩽ 0.05 when compared with control.
2.6 AsA and GSH levels
The non-enzymic antioxidants, viz., ascorbate (AsA) and glutathione (GSH) showed increasing trends with the increase in concentration and time of exposure (Fig. 6, A and B). Upon the imposition of oxidative stress, the existing pool of reduced glutathione (GSH) is converted to oxidized glutathione (GSSG) and glutathione biosynthesis is stimulated. The rate-limiting step for glutathione synthesis is thought to be gamma-glutamylcysteine synthetase, which is feedback regulated by GSH and is controlled primarily by the level of available L-cysteine [28]. The level of AsA increased maximum at 100 μM Cu after 18 h treatment in roots after 24 h of Cu treatment as compared to control. Whereas the GSH level increased maximum in roots at 50 μM Cu after 18 h and in leaves at 100 μM Cu after 24 h treatment as compared to control. Ascorbate (AsA) is found in great quantities in the plants in which it exhibits many antioxidative properties [29]. It can directly scavenge ROS (including hydroxyl and superoxide radicals and hydrogen peroxide) either non-enzymatically or enzymatically [30]. In the latter case, it is used as a substrate for the H2O2-splitting enzyme ascorbate peroxidase [31]. It can also indirectly act as an antioxidant by regenerating the membrane-bound α-tocopherol which is involved in the scavenging of peroxyl radicals and singlet oxygen [32]. In absence of the detoxifying role of antioxidant enzymes, both AsA and GSH showed enhanced activity. It might be possible to conclude that the two non-enzymic antioxidants might actively participate in ROS detoxification.
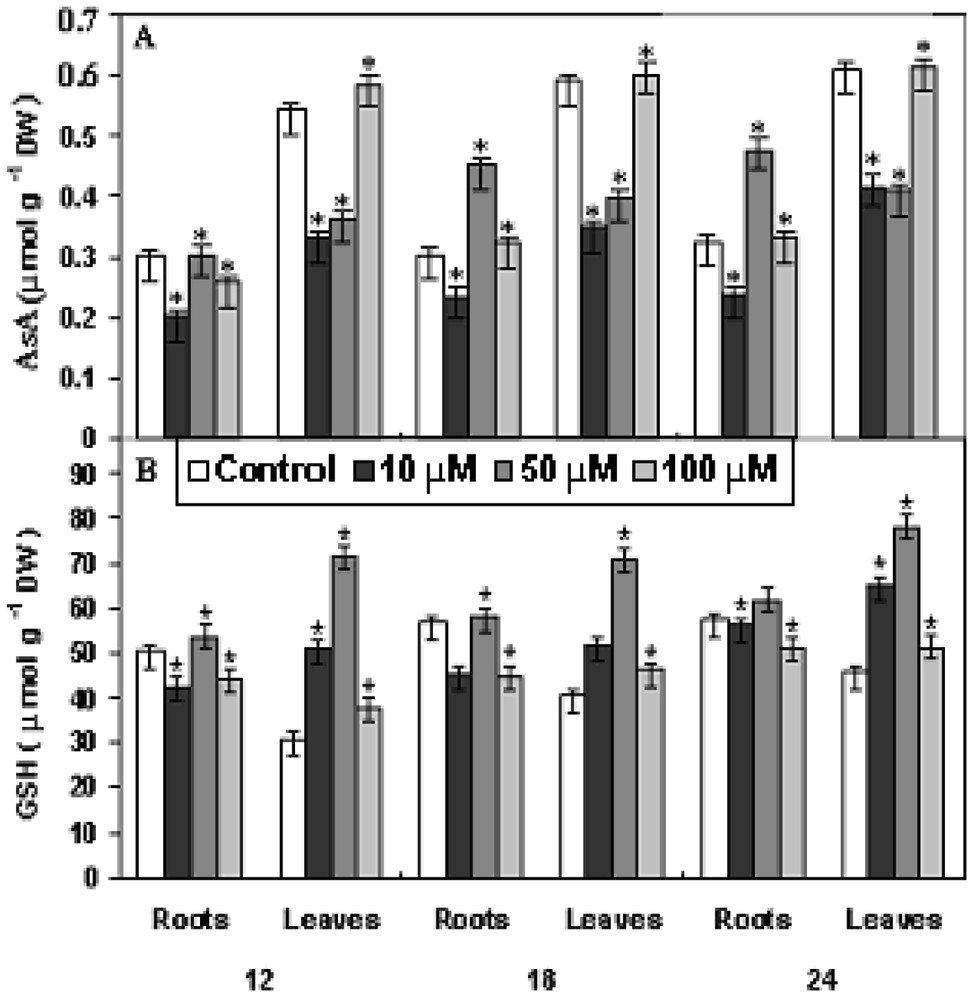
Changes in the levels of (A) ascorbate and (B) glutathione in P. stratiotes L. after 12, 18 and 24 h of CuSO4 exposure. The data presented are means of three replicates. ∗ represents the significance level at P ⩽ 0.05 when compared with control.
2.7 Protein content
A concentration–time dependent initial increase with a decrease in higher concentration of total protein was observed in Fig. 7. In both roots and leaves, protein content increases initially with maximum (upto approx. 80%) in leaves at 100 μM Cu after 18 h. However, after 18 h, the protein content gradually decline in both roots and in leaves at 50 μM Cu and 100 μM Cu as compared to control. Protein content in an organism is an important indicator of reversible and irreversible changes in metabolism, known to respond to a wide variety of stressors such as natural and xenobiotic [20,33]. In the experimental results, a significant inhibition of protein level after 18 h was visible. The inability of Pistia plants to synthesize protein after Cu exposure might have caused by acute oxidative stress induced by elevated concentration in plant cells, as also reported in Lemna fronds [20].
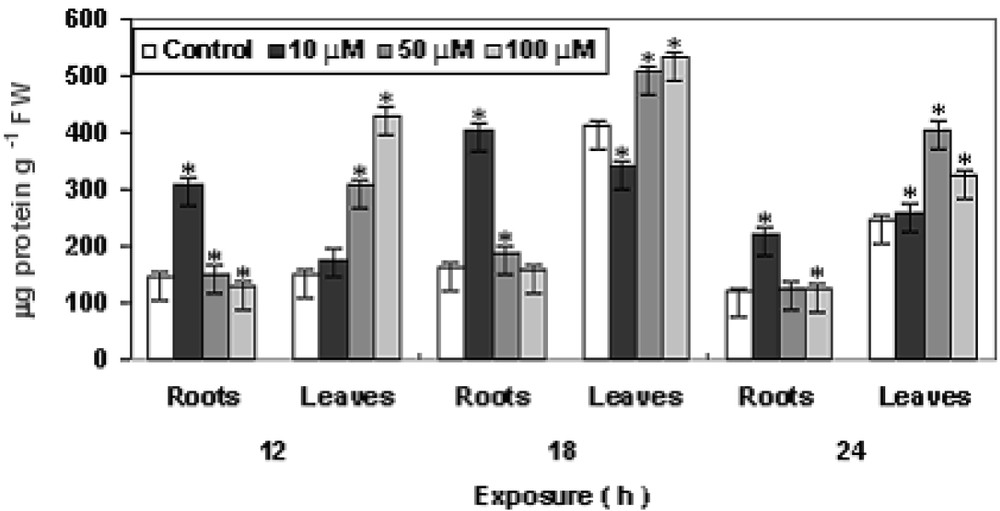
Changes in protein contents in P. stratiotes L. after 12, 18 and 24 h of CuSO4 exposure. The data presented are means of three replicates. ∗ represents the significance level at P ⩽ 0.05 when compared with control.
2.8 Transmission electron microscopy
The changes in cell ultrastructure as seen under transmission electron microscopy are shown in Fig. 8, a and b. In control leaves the chloroplasts were well elongated having clear stroma and thylakoids and mitochondria were globular in shape found adjacent to the cell wall which was quite distinct. As compared to this, in Cu treated leaves chloroplast and mitochondria became smaller in size with distorted structure, and appearance of large vacuole and indistinct cell walls were observed after 24 h of treatment, thereby affecting the photosynthesis and respiration that substantiated the biochemical lesions in Pistia plants. Similar observations were seen for other plants [34].

Transmission electron microscopy studies of an ultrathin section of leaves of P. stratiotes L. with or without CuSO4. a – untreated leaves (control); b – treated with CuSO4 at 100 μM. Both the observations were done after 24 h Cu treatment. Scale bar = 5 μm.
Hence the concentration–time dependant short term copper exposure had a remarkable effect on the physiology and biochemistry, induced oxidative stress in Pistia plants under controlled environment as evidenced by biochemical alterations, changes in antioxidant efficiency and ultra structural changes suggesting a possible mechanism of Cu phytotoxicity in water lettuce (P. stratiotes L.). Copper is able to trigger oxidative stress in the rooted aquatic macrophyte P. stratiotes L. explained by the increase in antioxidative protection during stress caused by elevated concentration of Cu, which suggests increased formation of ROS and probably is in parts responsible for the decline of the submerged aquatic macrophyte vegetation. But increase antioxidant levels helped the plants to cope with the stress under repeated metal exposure. This study further with respect to changes in expression of major antioxidative genes along with the regulatory proteins under Cu stress can reveal better understanding of copper tolerance and toxicity mechanisms in aquatic macrophytes.
3 Materials and methods
3.1 Plant material and treatment
Fresh samples of Pistia stratiotes L. were collected from uncontaminated ponds nearby Assam University, Silchar, India. Plants were brought carefully to the laboratory in clean plastic jars. Healthy plants were selected and then cultured in 1:40 (v/v) Hogland's Nutrient solution as suggested by Bassi and Sharma [35]. Plants were cultured in transparent plastic tumblers inside the growth chamber for 7 d prior to Cu treatment and white light with photon flux density of 52 μmol s−1 m−2 (PAR) was provided with 16 h photoperiod. Cu treatment in the form of copper sulfate (CuSO4) was given at concentrations 0, 10, 50, 100 μM and both leaves and roots were harvested after 12, 18 and 24 h.
3.2 Measurement of growth and copper analysis
Growth was measured in terms of root and leave biomass. 2 g of both root and leave tissues were dried at 80 °C separately for 48 h and weighed to measure the dry biomass. The dried tissues were digested with 5 ml HNO3 at 100 °C until the solution turned clear. The sample volume was adjusted to 20 ml with de-ionized water and the bioaccumulation of Cu content was measured using atomic absorption spectrometer (Perkin–Elmer 3110, Germany).
3.3 Estimation of total chlorophyll and carotenoid
The leaves (0.5 g FW) were ground in 10 ml of 80% acetone and allowed to stand overnight in dark at 48 °C, followed by centrifugation at for 5 min. Content of chlorophyll and carotenoid in the supernatant was determined according to Lichtenthaler [36].
3.4 Determination of malondialdehyde content
The level of lipid peroxidation, expressed as MDA content, was determined as 2-thiobarbituric acid (TBA) reactive metabolites. Plant fresh tissues (0.2 g) were homogenized and extracted in 10 ml of 0.25% TBA made in 10 ml trichloroacetic acid (TCA). Then extract was heated at 95 °C for 30 min and then rapidly cooled in ice. After centrifugation at for 10 min, the absorbance of the supernatant was measured at 532 nm. Non-specific turbidity was corrected by subtracting the absorbance value taken at 600 nm. The concentration of MDA was calculated using extinction coefficient of 155 m M−1 cm−1 [37].
3.5 Determination of hydrogen peroxide and superoxide radical content
H2O2 content was measured according to Sagisaka [38]. 1 g of tissue was homogenized in 5% trichloroacetic acid (TCA) and the homogenate was centrifuged at at 0 °C for 10 min. The reaction mixture contained 1.6 ml supernatent root extract, 0.4 ml TCA (50%), 0.4 ml ferrous ammonium sulfate and 0.2 ml potassium thiocyanate. The absorbance was recorded at 480 nm. The level of was assayed spectrophotometrically by measuring the reduction of exogenously supplied nitroblue tetrazolium (NBT) according to Doke [39]. Ten leaf discs (diameter 3 mm) were immersed in 2 ml of the mixture containing 0.01 M sodium phosphate buffer pH 7.8, 0.05% NBT and 10 mM NaN3 in a beaker (volume of 25 ml). After 60 min of incubation 1.5 ml of the reaction solution was transferred into a test tube and heated at 85 °C for 15 min. Then the solution was cooled and its absorbance at 580 nm was measured. NBT reducing activity (indicating generation) was expressed as the increase in A580 per hour per gram dry weight.
3.6 Measurement of antioxidant enzyme activity
The root and leave tissue was homogenized with phosphate buffer, pH 6.8 (0.1 M) in pre-chilled mortar and pestle. The extract was then centrifuged at 4 °C for 15 min at in a cooled centrifuged. The supernatant was used for the assay of POX (EC 1.11.1.7) and SOD (EC 1.15.1.1) and CAT (EC 1.11.1.6). The POX and CAT activities were assayed as per the method of Chance and Maehly [40]. The 5.0 ml mixture consisted of 3.0 ml phosphate buffer (pH 6.8), 1 ml (30 mM) H2O2 and 1 ml enzyme extract. The 3.0 ml reaction consisted of 0.1 M phosphate buffer (pH 6.8), guaiacol (30 mM), H2O2 (30 mM) and 0.3 ml enzyme extract. The rate of change of absorbance at 420 nm was measured. The POX and CAT activities were expressed as μmol (H2O2 destroyed) g−1 (f.m.) min−1. The assay of SOD was performed as per the method of Giannopolitis and Ries [41]. The 3 ml assay mixture for SOD contained 79.2 mM Tris–HCl buffer (pH 6.8), containing 0.12 mM EDTA and 10.8 mM tetraethylene diamine, bovine serum albumin (0.0033%), 6 mM nitroblue tetrazolium, 600 μM riboflavin in 5 mM KOH and 0.2 ml enzyme extract. The reaction was initiated by placing the glass test tubes between two fluorescent tubes (Philips 20W). By switching the light on and off, the reaction was started and terminated respectively. The increase in absorbance due to formazan formation was read at 560 nm. Under these conditions, the increase in the absorbance in the absence of enzyme was 100%, and 50% initial was taken as an equivalent to 1 unit of SOD activity.
The total soluble protein in the supernatant was determined as per the method of Bradford [42] using BSA as standard.
3.7 Metabolite determination
Extraction and estimation of GSH was performed according to Griffith's [43] method. The root tissue was homogenized in 5% (m/v) sulfosalicylic acid and the homogenate was centrifuged at for 10 min. A volume of 1 ml of supernatent was neutralized with 0.5 ml of potassium phosphate buffer (pH 7.5). Total GSH content was measured by adding 1 ml neutralized supernatent to a standard solution mixture consisting of 0.5 ml of 0.1 M sodium phosphate buffer (pH 7.5) containing 1 ml EDTA, 0.2 ml of 6 mM 5,5-dithiobis-2-nitrobenzoic acid, 0.1 ml of 2 mM NADPH and 0.1 ml of 1 U yeast GR Type III (Sigma Chemical, St. Louis, USA). Change in absorbance was measured at 412 nm and followed at until the absorbance reached 0.5 U. Ascorbate expression and estimation were carried out according to the method of Oser [44]. The reaction mixture contained 2 ml 2% Na-molybdate, 2 ml of 0.15 M H2SO4, 1 ml of Na2HPO4 and 1 ml root tissue extract. It was mixed and incubated at 60 °C in a water bath for 40 min, then cooled, centrifuged at 3000g for 10 min, and absorbance was measured at 660 nm.
3.8 Transmission electron microscopy
Root and leave tissues were fixed in a solution of 3% (w/v) glutaraldehyde in 100 mM potassium phosphate buffer (pH 6.8) for 2 h at 4 °C. Post-fixation, ultramicrotomy and staining were carried out at the Sophisticated Analytical Instrumentation Facility (SAIF), North Eastern Hills University, Shillong, India. Ultrathin sections were observed under a transmission electron microscope (Joel JEM-100 CX II, Akishima, Tokyo, Japan).
3.9 Statistical analysis
All the observations were done in triplicate, repeated thrice and the data represent mean ± standard error of mean (SEM). The results were subjected to ANOVA using GLM factorial model on all parameters. The data analyses were carried out using statistical package, SPSS (ver. 10: SPSS Inc., Chicago, IL, USA). In figures, the values are marked as ∗ (asterisk) for the significance level as compared to control.
Acknowledgements
R.K. Upadhyay is grateful to the University Grant Commission (UGC), New Delhi, Government of India, for the award of UGC Research Fellowship (Award No-AUK-204/12/2004/7816-66).