1 Introduction
Crop protection is essential to safeguard agricultural production from weeds, pathogens and animal pests. Among the latter, some species are also vectors of pathogens. This is the case with aphids which are a serious problem for agriculture despite being a relatively small insect group (4000 species in the world, around 600 in France) compared to 10,000 species of grasshoppers, 12,000 species of geometrid moths and 60,000 species of weevils. The 4000 aphid species live mostly in temperate regions where they are colonizing 25% of the existing plant species [1]. However, only about 100 species have successfully exploited the agricultural environment to the extent that they are of significant economic importance [2].
Aphids weaken their host plants in diverse ways. First, as phloem-feeders, they are diverting for their own profit the nutrients necessary to plant growth and reproduction. Second, during the feeding phase, they inject saliva that could be phytotoxic. Third, aphids transmit numerous viruses: nearly 50% of insect-borne viruses (275 out of 600) are transmitted by aphids [3,4]. Finally, sooty moulds (black filamentous saprophytic ascomycetes) frequently grow on aphids’ honeydew and hinder photosynthetic activity.
Generally speaking, it is very difficult to give a precise assessment of the potential economic losses due to aphids, first because of the tremendous between-year variation in their population size and in the crop areas damaged, second because of the diversity of crops and agricultural conditions. An exception includes the introduction in 1986 in the USA of the Russian wheat aphid, Diuraphis noxia, a native from the steppe areas of the southern CEI (former USSR). It subsequently spread rapidly throughout wheat-producing regions of the western United States where it caused hundreds of million dollars losses in wheat and barley production, through reduced yields and pesticide treatment costs. Annual direct yield losses peaked at $274 million in 1988 and dropped to less than $10 million by 1993 [5].
Due to their paucity in tropics and subtropics, aphids are certainly of higher economic importance in temperate than in tropical crops. For example, aphids are considered only as minor pests in rice [6]. In contrast, an analysis of data gathered by Hill [7] indicates that, among the 45 major insect pests on the 6 main food crops of temperate climate (maize, wheat, potatoes, sugar beet, barley and tomatoes), 26% belong to aphids (Fig. 1). In Europe, Wellings et al. [8] indicate that direct damage by aphids is responsible for mean annual losses of 700,000 t of wheat, 850,000 t of potatoes and 2,000,000 t of sugar beet. Tatchell [9] gave for Britain mean percentages of direct losses from aphids of 8–16% in pea, 10–13% in wheat and 5% in potato.
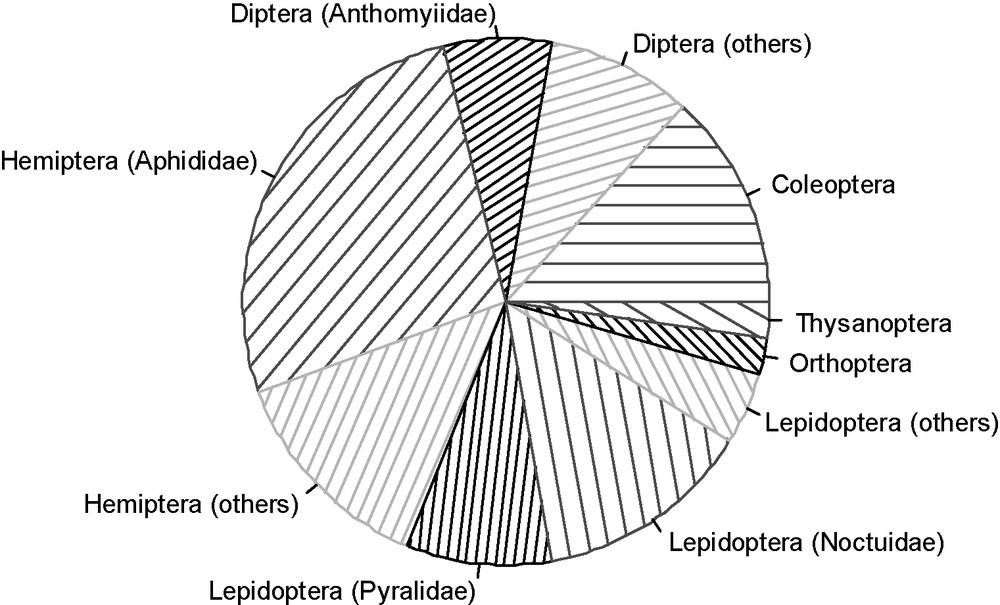
Repartition of the taxonomic order of the 45 major insect pests recorded by Hill [7] for the six main food crops of temperate climate (maize, wheat, potatoes, sugar beet, barley and tomatoes – FAO stat 2007). Hill [7], in his book “Agricultural insect pests of temperate regions and their control”, defined a major insect pest as “a serious pest of a crop (or crops) in a restricted locality or are economic pests over a large part of the distributional range of the crop (or crops). Thus, the species regarded as major pests required controlling over a large part of their distributional (geographical) range, most of the time”.
Many of the 275 viruses transmitted by aphids cause diseases of major economic importance. Thus, the indirect damage that aphids cause through virus transmission often far exceeds their direct impact on crops. In cereal crops, Barley yellow dwarf disease (BYDD) is worldwide the most widespread viral disease, due to Barley yellow dwarf viruses (BYDV) and/or Cereal yellow dwarf viruses (CYDV) [10]. A survey of yield losses due to BYDD in early sown barley fields in France indicates losses ranging from 0 to 80% with a mean of 20% (Fig. 2). Sugar beet crops are affected in Europe by several aphid-transmitted yellowing viruses (e.g., Beet yellows virus (BYV), Beet mild yellowing virus (BMYV)) that can decrease sugar yield up to 49% [11].
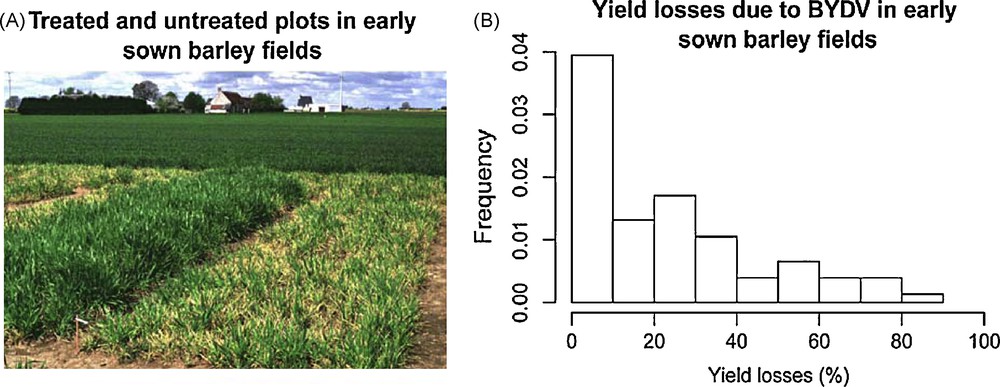
From 1989 to 2002, several field experiments in 15 locations in the northern part of France were monitored to investigate Barley yellow dwarf disease (BYDD) epidemiology. The data set obtained is representative of a wide range of climatic conditions and aphid infestation levels (from 2 to 100% of plants infested by at least one aphid). A: In each experiment, plots were sown from mid-September to mid-October with winter barley in a randomised complete block design with four replications and two factors (untreated receiving no insecticide spray and treated receiving two insecticide sprays). The individual plot size was 3 × 11 m. In the untreated plots, spontaneously colonised by aphids, the proportion of plants infested by Rhopalosiphum padi was monitored at intervals varying between 5 and 14 days from crop emergence until about the end of November. In treated plots aphid populations were totally controlled by two insecticide sprays with a pyrethroid (Decis®) ensuring a complete protection against BYDD. B: Distribution of the percentage yield losses due to BYDD in early sown barley fields (n = 76). Percentage yield losses are assessed as the ratio of the yield obtained in untreated plots to that obtained in treated plots.
In vegetables and fruits, viruses are often associated with huge losses of production quality with many unmarketable products. In field-grown vegetables, a synthesis by Tomlinson [12] over 28 countries with temperate climate revealed that the five most economically important viruses are transmitted by aphids (Cucumber mosaic virus (CMV), Turnip mosaic virus (TuMV), Potato virus Y (PVY), Lettuce mosaic virus (LMV) and Papaya ringspot virus (PRSV)). The two most common viruses of potatoes, PVY and Potato leaf roll virus (PLRV), are transmitted by aphids and are of particular concern to seed potato producers [13]: in France, if more than 1% (class ‘super elite’), 2% (‘class elite’) or 5% (class A) of them are infected by PLRV and/or by PVY, the seed class must be downgraded. In citrus, the most damaging diseases of the last decades is caused by the aphid-borne Citrus tristeza virus [14] and in stone-fruit crops (e.g., peach, apricot) the aphid-borne Plum pox virus (PPV), is a major problem responsible for sharka disease [15].
It is their ability to rapidly exploit the ephemeral habitats constituting most agricultural landscapes that makes aphids serious pests, causing important losses in yield and/or quality in most arable, horticultural and fruit crops. This ability results from: (i) their high reproductive potential; (ii) their dispersal capacities; and (iii) their adaptability to local survival. First, although not very fecund (40–100 offspring/female in average), aphids have a high reproductive potential due to their long period of parthenogenesis (all individuals are females for much of the year) combined to a short generation time. This leads to high daily intrinsic rates of increase (rm) ranging from 0.1 to 0.4 according to temperature. For instance, populations of the aphid Sitobion avenae (rm = 0.3 at 20 °C from data of Simon et al., 1991 [16]) are multiplied by 2 every 55 h. Second, in response to short-term changes in population size and host quality (overcrowding, plant senescence), aphids produce winged morphs able to disseminate by air over large distances [17]. Quantities of winged aphids immigrating to fields could be very large: mean winged S. avenae input in wheat fields during June was between 5 × 105 and 1.5 × 106 individuals per hectare [18]. Third, life-cycle polymorphism allows many aphid species to optimize locally their survival during winter: this life cycle polymorphism is strongly affected by climate [19]. Cyclical parthenogenetic lineages are preponderant under severe winter conditions [20]. They produce sexual morphs in autumn, which mate and lay low temperature-resistant diapausing eggs while obligate parthenogenetic lineages overwinter as mobile parthenogenetic individuals under mild winter condition. In most temperate areas, both types of aphid life cycle coexist and the local balance between them has been shown to correspond to an evolutionary stable strategy [21].
All these characteristics can lead to spectacular aphid build-up, but not every time and everywhere because of multiple interacting demographic factors, one of them being the action of numerous natural enemies [22]. This variability of build-ups renders aphid management particularly challenging in agriculture. In this article, we will now detail the mechanism leading to yield losses. Then the main control strategies are reviewed in the light of an integrated pest management approach. Several topics tackled in this article are exemplified in cereal aphids, especially Rhopalosiphum padi which is the most important vector of the viruses responsible of Barley Yellow Dwarf Disease (BYDD) in autumn-sown cereals in Europe [23].
2 Mechanisms leading to aphid damage
2.1 Damage caused by sieve drain
Sieve diversion by numerous aphids affects plant physiology in different ways, depending on the plant growth stage when infested. In fruit trees, the most common reaction is twig stunting because of reduced growth. Aphid feeding on flowers (e.g., Myzus persicae on peach trees) can lead to bad fructification (flower abortion). If aphid colonies develop later, fruits can be smaller. Most of these injuries are ‘asymptomatic’ [24]: for example some cereal aphids, in the absence of viruses, decrease root proliferation, size and yield of cereals without any other particular visible symptom. When aphids are numerous, these kinds of damage are often increased by honeydew excretion, which covers the leaf cuticle and is colonized by sooty moulds, thus hindering photosynthesis and affecting the marketability. On cotton crops, the main cause of the “sticky fiber” symptom is the penetration of honeydew produced by Aphis gossypii in open mature boll [25].
The global intensity of injury roughly depends: (i) on the quantity of aphids on the plant (or the organ) at each developmental stage of the plant; and (ii) on the sensitivity of each plant stage to sieve diversion. For example, wheat yield will be more especially lowered when the crop is colonised early by the aphid S. avenae: a strong aphid infestation at heading or flowering lowers the number of grains per ear, whereas later infestation (e.g., milky ripeness…) only involves a decrease of grain weight [26]. For this aphid species, a damage function has been established [27] that forecasts quite well the final loss as a sum of the damage done at each growth stage of wheat (Fig. 3).
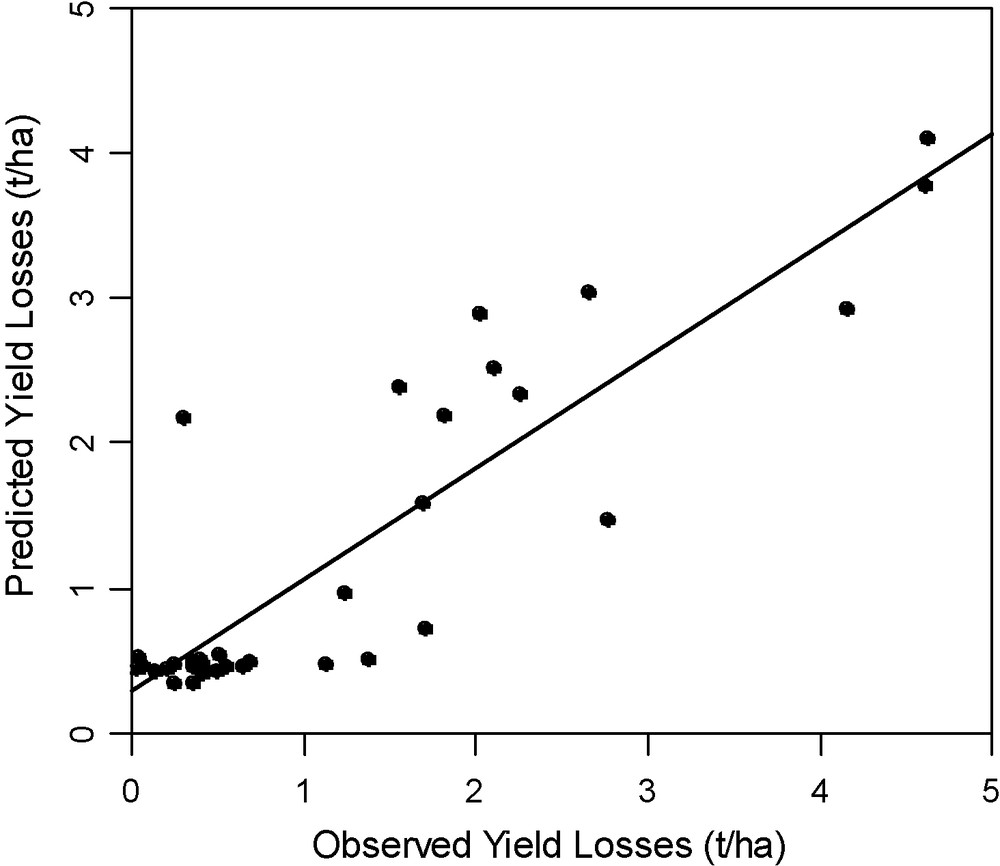
Validation of the S. avenae wheat damage function, from Vialatte et al. [27]. X-axis: measured yield losses due to the aphid S. avenae in spring on wheat in different French regions (several trials pooled). Y-axis, yield losses estimated by a damage function cumulating effects of aphids at different growth stages of wheat: . : total damage; αi: damage coefficient at growth stage i; IRi: aphid number at growth stage i.
However, in some cases, for instance the aphid Acyrthosiphon pisum on peas, complex mechanisms are involved in the plant response to the action of the aphids. They only lead to the stimulation of the growth of nodes with no measurable yield losses: seed weight per plant or nitrogen content was not reduced in any of the experiments, leading to the conclusion that the natural local dynamics of the aphid would not involve damage of economic importance [28].
2.2 Symptomatic damage due to plant reaction to aphid feeding
In numerous cases [29], plants react to aphid feeding by leaf decolouration (e.g., the Russian wheat aphid, D. noxia), necrosis (e.g., Schizaphis graminum on sorghum and Macrosiphum euphorbiae on potato), leaf or/and fruit deformations (Dysaphis plantaginea on apple trees). In some other cases, aphid feeding strongly disturbs cell multiplication around the feeding point, leading to plant tissue proliferation (neoplasms) forming galls. The latter cases are mainly observed with fundatrices hatching from overwintering eggs. Galls may be open (pseudo-galls, e.g., R. padi on its primary host Prunus padus) or closed (‘true galls’) enclosing the aphid colony, like those of several species of Pemphigus living on poplars, and those of the elm-tree galling aphid Eriosoma lanuginosum (Fig. 4). These galls have an important role in the biology of the aphid species that induce them: they are shelter, source of nutrients and they maintain a favourable microclimate around aphid colonies. Do they really protect the aphids against their natural enemies? In many cases, the gall does not protect completely the aphids from predators [30]. In some cases, aphids protect the gall: indeed, a number of galling aphid species are known to produce specialised “soldier” forms, that are sterile nymphs with defensive features used to defend the gall from invasion to a certain extent, as shown for P. spyrothecae [31,32] and other Pemphigidae [33].
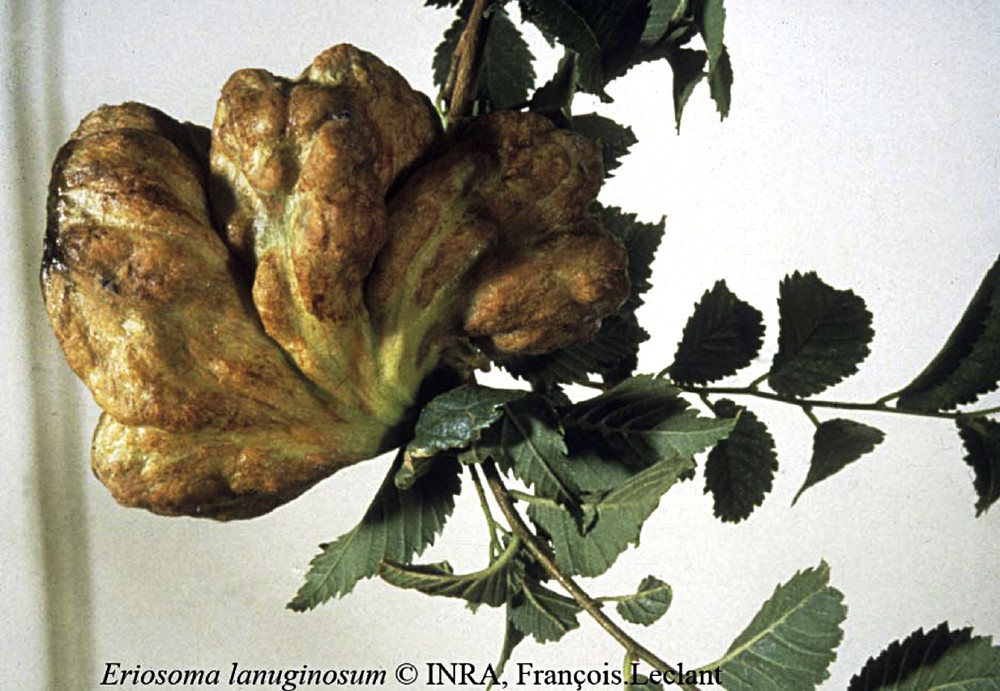
A true gall, from the elm-tree aphid Eriosoma lanuginosum.
Do those galls cause losses? Certainly on fruit trees, for example in cherry trees, pseudo galls of the aphid Myzus cerasi are most often very numerous, provoking early leaf fall and consequently reducing cherry size.
The biochemical processes of gall formation are only partly known: when feeding, aphids inject two types of saliva into the plants, an aqueous one and a more gelatinous one. Both contain different chemical compounds likely to interact with plant cell physiology in variable quantities depending on the aphid species. Several enzymes like pectinase, catalase, peroxydase seem to be responsible for necrosis, stunting, and vein-clearing symptoms [24]. Moreover, cecidogenic compounds like tryptophan derivatives (β-indol acetic acid) have been identified in low concentration from the saliva of at least two gall-inducing aphids, the grape phylloxera Daktulosphaira vitifoliae and the woolly apple aphid Eriosoma lanigerum [29]. However, gall formation does not seem to be a general response to injected aphid biochemicals: most gall-forming aphids do not seem to produce vegetal hormones [34] and the search for aetiological agents of plant deformations by aphids is still an ongoing process.
2.3 Damage due to virus transmission
During their replication, plant viruses provoke histological and cytological effects in their hosts causing different physiological disturbances. Their consequences are highly dependent on the severity of the virus strain(s) inoculated. Some viruses, under appropriate conditions, may infect a plant without producing any symptoms. Others may lead to the rapid death of the whole plant. Between these extremes, a wide variety of damage can be observed [35]. As an example, we give below the physiological steps of barley infection by a severe PAV strain of BYDV and their consequences for the crop.
BYDV particles inoculated by aphids during sieve punctures circulate in the phloem vessel and infect companion cells nearby where they replicate. The virus particles are spread through the phloem vessel to roots and then to leaves. Companion cells react to infection by producing calloses, progressively stopping up the phloem vessels and consequently hindering sieve flow. This involves starch accumulation in the leaves (three times more than in an uninfected plant on average). As a result, the dry weight of the plant increases, chlorophyll concentration decreases and consequently photosynthesis is inhibited, first affecting root growth [36]. Depending on the moment and severity of infection, damage includes mortality of young plants, weak tillerage, bad heading and reduced grain number and size.
3 Chemical control of aphids
3.1 Historical overview
Before the Second World War, the only insecticide really efficient against aphids was ‘natural’ nicotine, extracted from tobacco leaves and sold as sulphate. Nicotine was registered in Europe until the middle of the 1990s. It kills aphids that come into contact with the product and its action is not persistent at all. Nicotine is extremely toxic to vertebrates and consequently, no longer available. The first organochlorinated insecticide, DDT, was discovered in 1939. After the war, chemical control of aphids made rapid progress with other organochlorinated compounds in the late 1940s, organophosphorous in the 1950s, carbamates in the 1960s and pyrethroids during the 1970s. The latter are still by far the most used for spraying on plants.
During this period (1950–2000), the dosage, as well as the toxicity, of aphicides to vertebrates have markedly decreased: doses of active ingredient per hectare have been divided by 10 on average, and LD50 (lethal doses for 50% of the target population of vertebrates) have been multiplied by 10. In the same period, some more selective insecticides have been registered, especially in the groups of organophosphorous (e.g., phosalone) and carbamates. However, their specificity is relative and restricted to some rare compounds. The popular pyrethroids are not selective.
Since the beginning of the intense use of aphicides, resistant clones of different aphid species (mainly M. persicae and A. gossypii) to one or more families of insecticides have been selected in the most treated agricultural areas in the world. By the mid 1980s, around 20 aphid species in the world had developed resistant clones to one or more insecticides [37]. For example, at the moment, at least 8 aphid species among the most noxious to arable crops, vegetables and/or fruit trees are resistant to one or to several insecticide families in France (R. Delorme, pers. comm.). Insecticide resistances are the most diverse and frequent in the peach-potato aphid, M. persicae. In this aphid specie, at least four mechanisms have been shown to cause insecticide resistance and cross-resistances: esterase E4 and F4 amplification, acetylcholine esterase (AChe) modification, mutation of GABA receptor (gene Rdl) and mutation on Na+ canal (gene Kdr) [38]. In spite of: (i) aphids high migratory capacities; and (ii) large and intense insecticide pressure, aphid (e.g., M. persicae) populations have never been invaded by resistant clones, probably because there is a ‘cost of resistance’: resistant clones should be counter-selected locally when there are no insecticide treatments [38].
At the end of the 1980s, a new insecticide family arose, the neonicotinoids (chloronicotiniles and thianicotiniles), that are strongly plant systemic: they are transported in the different parts of the plant by xylem and phloem vessels from the treated area of the plant. Their main advantage is to be usable as seed treatment. In this case, aphids and other phloem feeding insects are intoxicated after their first sap ingestion. This makes this kind of insecticide very efficient for blocking some virus spreads [39]. Moreover, natural enemies of aphids are not directly targeted (but they can suffer from the lack of prey or by eating intoxicated prey).
In most European countries, neonicotinoids are authorised as seed treatments for the control of aphids as virus vectors in some annual crops (e.g., barley, wheat and sugar beet in France). Their major drawbacks are to generalise systematic insecticide use, because it is difficult, if not impossible, to forecast the aphid risk when seeds are ordered (at least 2–3 months before the sowing).
Finally, there are specific insecticide treatments for aphid overwintering eggs in orchards: they are efficiently killed (asphyxiated) by sprays of petroleum-derived oils on trees in winter. These oils have been used since the 1930s [40].
3.2 Limits of chemical control
There are many limits to the use of insecticides for aphid management in agriculture. First, they can be too expensive regarding the yield loss avoided. Second, if insecticides efficiently prevent direct damage that is, in most cases, roughly proportional to the size of aphid populations at the susceptible growth stage of the crop, this is not always the case for indirect damage. Insecticide efficiency against damage due to aphid-borne viruses indeed depends mainly on the mode of transmission of the virus and on the pattern of virus spread [41,42]. Two main transmission modes can be distinguished [43]. Non-persistent viruses are transmitted non-specifically by a large number of aphid species after very brief probes (seconds to minutes) and are retained over a few hours in the vector. Conversely, persistent viruses are transmitted much more specifically after a long inoculation access period (1–48 h) and are retained in the vector during its whole life. In their review, Perring et al. [42] indicate that among the reported successes of controlling virus spread using insecticides, 78% (n = 119) were with persistently (or semi-persistently) transmitted viruses. These cases include, for example, major viruses of cereals (BYDV and CYDV), of potato (PLRV) and sugar beet (BYV and BMYV). In contrast, 66% (n = 48) of the reported failures involved non-persistently transmitted viruses. For these viruses, which represent 200 of these 275 viral species transmitted by aphids, insecticides are often ineffective because viruses are transmitted to plants in a very short time during superficial stylet punctures, by several aphid species which, in most cases do not develop on them. In some cases, insecticides can even speed up virus spread by increasing vector activity [44]. Exceptions to this trend have been observed especially using pyrethroids, oils and behaviour-modifying chemicals [42]. Regarding the pattern of spread, the relative proportion of primary infection events (the virus is brought into the field by insects already infective) and secondary spread events (the virus is spread from an infected to a healthy plant within the field) in an epidemic is important. When the primary infection process overcomes secondary spread and especially when the proportion of viruliferous aphids that enter the field is high, insecticide treatment is rarely efficient. In contrast, applications of insecticides are more efficient if the secondary spread dominates. Others reasons for inefficiency include the spread of insecticide resistance (e.g., peach–potato aphid M. persicae in southern France [45,38]) and improper uses.
Another limit to insecticide use is that several insecticides have been banned by law since 1999 in several countries (e.g., due to the European Union directive 91/414/CE), and farmers are now lacking efficient treatment for some specific use, such as in France against the woolly apple aphid E. lanigerum. Finally, without looking to deny the benefits of pesticides to mankind [46], the nowadays largely recognised hazards of pesticides both to health and environment imply that crop protection against aphids must be thought according to Integrated Pest Management (IPM) rules where, theoretically, insecticides are used as the last resort. IPM can be indeed defined as “an ecosystem approach to crop production and protection that combines different management strategies and practices to grow healthy crops and minimize the use of pesticides”. Interestingly for this special issue, an article by Stern et al. published 50 years ago [47] on the control of the spotted alfalfa aphid Therioaphis trifolii is often referred as one of the works on which the IPM concept was elaborated [48,49].
Below we shall see the main control methods available for managing aphids according to IPM rules. These methods include the use of host plant resistance, biological control and cultural methods which all offer powerful levels of action to manage aphid populations. For example, the area wide decline of the Russian wheat aphid documented earlier in this article is attributed to the use of resistant varieties combined with a gradual increase in the populations of native natural enemies [5]. However, first, we will introduce the decision-making tools devoted to making rational use of insecticides. Using these tools is the first step towards the adoption of IPM and its integration across the three hierarchical ecological scales: species/populations, communities and ecosystems [50].
4 Forecasting aphid risk for spraying only when necessary
The wide range of yield losses caused by BYDD, from 0 to 80% (Fig. 2), illustrates the variability from field to field and year to year of most aphid build-ups. Faced with this large variability and its apparent unpredictability, farmers are tempted to spray systematically according to calendar or crop phenology even if, in many cases, the cost of damage by the pest is less than the cost of control. This economical concept, introduced by Stern et al. [47], provides the basis for a decision framework for pest control. In return, it requires forecasting aphid risk a few days to months ahead.
Variability in aphid build-up and the worldwide characteristic of the aphid problem certainly contribute to the burgeoning literature devoted, as early as in the beginning of the 1980s, to aphid risk forecasting. Table 1 gives some references describing models aiming to improve aphid control. For an overview of this topic, the reader is referred to the chapters “Monitoring and forecasting” [72] and “Decision Support Systems” [73] of the book “Aphid as crop pests”. Instead here, following a rapid introduction to binary decision rules, we will describe the principles of a decision support system (aphi.net®) developed by INRA to forecast outbreaks of BYDV epidemics and currently distributed in France by Bayer CropScience.
A selection of forecasting models for aphid and virus control published since 1981.
Crop | Aphids/Virus targeted | Country | References |
Cereals (winter wheat) | Sitobion avenae, Metopolophium dirhodum, Rhopalosiphum padi | Netherlands | [51,52] |
Cereals (winter wheat) | Sitobion avenae, Metopolophium dirhodum | England | [53,54] |
Cereals | Sitobion avenae, Metopolophium dirhodum, Rhopalosiphum padi | Denmark Baltic countries | [55] |
Cereals (winter wheat) | Sitobion avenae | Sweden | [56] |
Cereals (winter barley) | Rhopalosiphum padi / Barley yellow dwarf virus | France | [57,58]; this article |
Cereals (winter wheat) | Sitobion avenae | France | [59,60] |
Cereals (wheat) | Rhopalosiphum padi / Barley yellow dwarf virus | Australia | [61] |
Cereals (winter wheat and barley) | Rhopalosiphum padi / Barley yellow dwarf virus | United Kingdom | [62,63] |
Cereals (winter wheat) | Sitobion avenae | France | [64] |
Cereals (wheat) | Diuraphis noxia | USA. | [65] |
Sugar beet | Myzus persicae/Beet yellows virus, Beet mild yellowing virus and Beet chlorosis virus | United Kingdom | [66] |
Hops | Phorodon humuli | Czech Republic | [67] |
Spring beans | Aphis fabae | United Kingdom | [68] |
Lupins | Several aphid species/ Cucumber mosaic virus | Australia | [69] |
Potatoes (seed production) | Several aphid species/Potato virus Y | Sweden | [70] |
Potatoes (seed production) | Several aphid species/Potato virus Y | Switzerland | [71] |
4.1 Binary decision rules and their analysis
When making a decision on whether to apply a pesticide, a farmer has to choose between three possible control strategies. These are: (i) to spray prophylactically; (ii) to never spray; or (iii) to spray according to the recommendations of tactical models [74]. Tactical models are designed to advise farmers on the need, and sometimes timing, of applying crop protection measures [49]. The simplest tactical models are decision rules designed to provide farmers with binary advice: “Yes, treatment is needed” or “No, it's not worth the trouble”. Such decision rules associate a risk indicator (I) and a decision threshold (Is): in a field where I ≥ Is, a treatment is recommended and unnecessary if I < Is.
Various decision rules can be defined according to the decision threshold (Is) selected, but a single rule would be economically optimal. The accuracy of indicators discriminating between two alternatives (e.g., the cases T+ “a treatment is needed” and the control T− “a treatment is not justified”) can be assessed by their sensitivity (Se, probability of true positive decision, e.g., probability that a decision rule recommends a treatment when it is really needed) and their specificity (Sp, probability of true negative decision, e.g., probability that a decision rule does not recommend a treatment when it is really not necessary) [75,76]. Se and Sp, defined as p(I ≥ Is|T+) and p(I < Is|T−) respectively, are independent of the prevalence (Prev) of the cases but dependent of the decision threshold Is. The effect of this threshold is commonly described with Receiver Operating Characteristic (ROC) curve [75] which is a plot of Se against 1–Sp for all possible values of Is. ROC curves are a valuable tool for deriving optimal value of Is as ROC curve analysis is directly related to cost/benefit analyses [75]. It is also a valuable tool for comparing the overall accuracy of several indicators using, for example, the Area Under the ROC Curve (AUC) [76].
4.2 An example of binary decision rule for BYDV management
To illustrate these points, the principles of the decision support system aphi.net® are now detailed. This decision tool provides farmers with binary advice on whether to treat or not against BYDV by comparing the value of a risk indicator, assessed at the field scale from an early estimate of the proportion of barley plants infested by aphids, with a decision threshold. The risk indicator is assessed by coupling a population dynamic model of BYDV vectors with a model relating vector dynamics to BYDD yield losses. These two models are first introduced before describing how an optimal financial decision threshold is derived.
4.2.1 A population dynamic model of BYDV vectors
The model forecasts the population dynamics of the aphid Rhopalosiphum padi in barley fields during autumn [57]. It is based on a relationship between the growth rate of R. padi populations and temperature fitted on field data representing a wide range of agricultural, climatic and aphid infestation conditions. The model predicts the probability distribution of the area under the curve of the percentage of plants infested by R. padi (variable thereafter denoted A) from an early assessment of the proportion of plants infested by aphids. (i.e., at growth stage 1 or 2 leaves – when insecticide spray against BYD can still be applied). The Bayesian framework used allows one to account explicitly for sampling errors and uncertainty in model parameters caused by the numerous factors acting on aphid population dynamics under field conditions. Validation performed on a independent dataset and using 20-year average temperatures indicates a good fit between observed (Aobs) and predicted (Apr) values of A (r2 = 0.85) with 84% of Aobs values included in a 90% confident interval of Apr.
4.2.2 Linking vector dynamics and BYDV yield losses
Using a logistic regression model, the probability that an insecticide is needed to control a BYDD epidemic was related to the area under the curve of the percentage of plants infested by R. padi in autumn [74]. This probability is thereafter denoted p(T|A). Sixty-four field experiments were used (details on the experimental design are provided in the legend of Fig. 2) and split into 2 groups according to the economic threshold justifying an insecticide spray against BYDD. In the French economic context, this yield loss threshold was set to 0.5 t/ha (Ctrt). When the yield losses due to BYDD are greater than this threshold, an insecticide application is expected to give a positive net return. The first group contained the experiments with yield losses ≥ Ctrt (37 “cases” fields). The second group contained the experiments with yield losses < Ctrt (27 “controls” fields). A binary variable corresponding to the need to apply insecticide was defined accordingly and shown to be highly significantly linked to the autumnal population dynamics of R. padi.
4.2.3 Derivation of an optimal binary decision rule
In all, the probability that a treatment is needed in a given field, p(T), can be estimated using the law of total probability from the logistic regression model which provides an estimate of p(T|Apr) and from the predictive model of R. padi dynamics which provides an estimate of p(Apr). The probability p(T) is a risk indicator on which a tactical model providing binary advice to farmers can be set up. The empirical ROC curve of the BYDD risk indicator based on the assessment of p(T) on 53 independent field experiments is plotted in Fig. 5A. This same ROC curve fitted to a binormal model is also figured [77]. These ROC are always closer to the left and upper axes of the graph than the ROC curves of an alternative indicator deciding whether to spray or not simply according to the value of the field assessment of the proportion of plants infested by aphids necessary to estimate p(T). This indicates that p(T) is a more accurate risk indicator than this simpler field sampling alternative. The calculation of the AUC of both indicators confirmed this result: 0.88 for p(T) (Fig. 5C) versus 0.8 for the alternative indicator (Fig. 5B). It emphasized the added value of the modelling framework proposed.
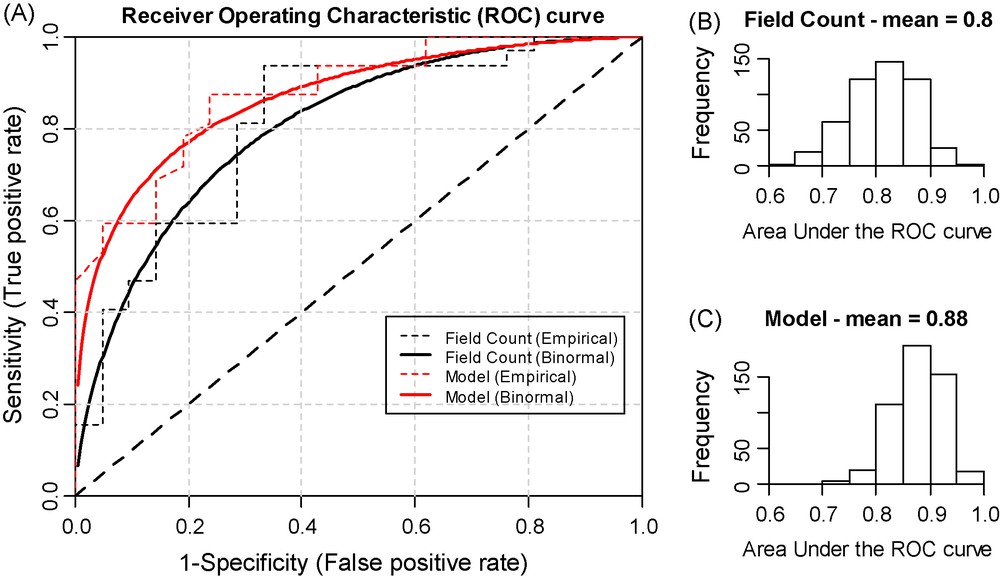
A: Empirical and binormal Receiver Operating Characteristic (ROC) curves showing two risk indicators of BYDD management. The first indicator, “Model”, estimated p(T), the probability that a treatment is required, by coupling a population dynamic model of BYDV vectors with a model relating vector dynamics to BYDD yield losses. This ROC curve is compared to the one of an alternative indicator “Field count” deciding whether to spray or not using the value of the early assessment of the proportion of plants infested by aphids. An indicator characterized by a ROC curve that comes near to the point (0.1) (where sensitivity and specificity equal 1) is a near-to-perfect indicator. In contrast, the ROC curve of an indicator unable to discriminate cases and controls shows a straight line joining the (0.0) point to the (1.1) point (the “no discrimination” line). B: Probability distribution of the Area under the ROC Curve (AUC) of the “Field count” risk indicator. The closer to 1 the AUC of an indicator is, the better this indicator is. C: Probability distribution of the AUC of the “Model” risk indicator. For each plot, ROC curves and AUC were assessed on 53 independent field experiments similar to that described in Fig. 2A using the R software (http://cran.r-project.org/) and the package “visualization”. This package implements a bootstrapping method from which the distribution probabilities of AUC are obtained.
As discussed previously, defining a decision rule requires choosing a risk indicator, here p(T), but also a decision threshold thereafter denoted S. ROC curve theory can be combined with cost/benefit analyses to derive the value of S (Sopt) minimising the mean annual cost of BYDD management, CDR:
4.2.4 Financial benefit of using the BYDV decision rule over systematic spraying strategies
In Fig. 6, CDR(Sopt) is plotted as a function of Prev and compared to the annual cost of BYDD management according to: (1) a “Never spray” strategy (CNS = Prev.Cmaj + (1–Prev).Cmin); (2) a “Systematic spray” strategy (CSS = Ctrt); and (3) a perfect decision rule that always recommends a treatment when necessary and never when it is not needed (Se and Sp of such a decision rule are equal to 1 and thus (CP = Prev.Ctrt + (1–Prev).Cmin). In the range of prevalence 0.02 to 0.78, the optimal decision rule derived (p(T),Sopt) always decreases the annual cost of BYDD management. Outside of this range, using the model is just as costly (but no more) as using a “Never spray” strategy (when Prev < 0.02) or a “Systematic spray” strategy (when Prev > 0.78). Compared to the “Systematic spray”, the decrease of the annual cost of BYDD management is at least 20% (i.e., 0.1 t/ha) for Prev < 0.34. Moreover, in this range of prevalence, the specificity of the model is at least 0.35: spraying according to model recommendation avoids at least 35% of unnecessary treatment.
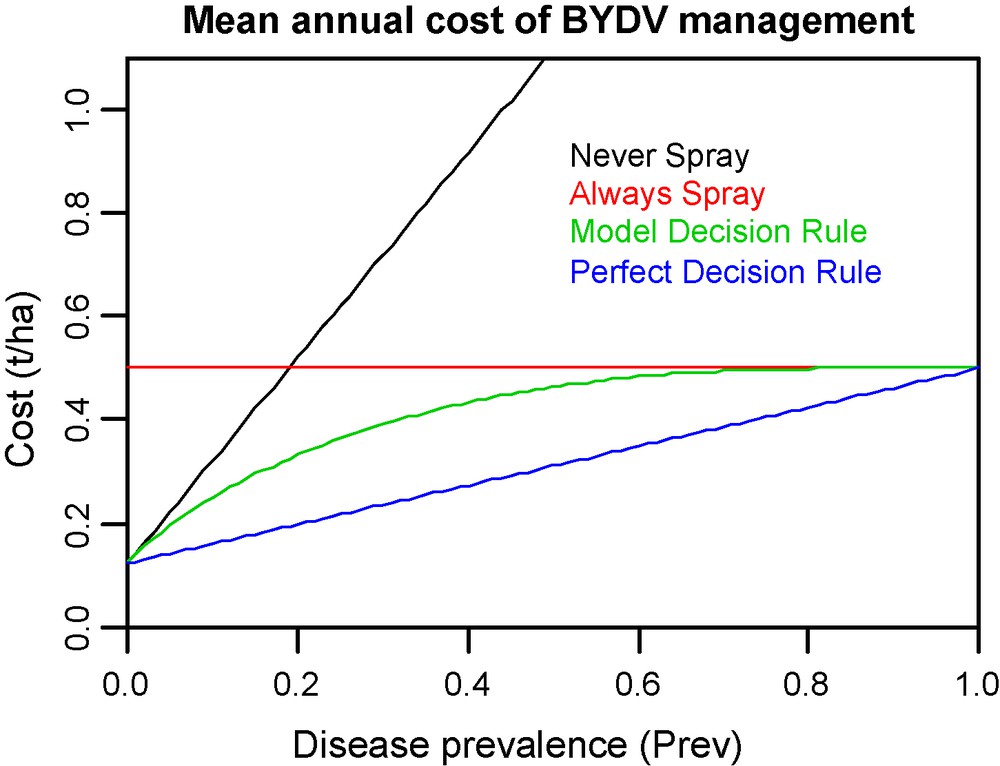
Mean annual cost of BYDD management as a function of disease prevalence according to four control strategies. The first two are systematic strategies consisting of “Never spray” or “Always spray”. The third strategy is to spray according to a model, the binary decision rule (p(T),Sopt) described in this article. The last strategy is to spray according to a perfect decision rule. Costs are expressed in yield loss equivalents (1 t = 1000 kg). Disease prevalence is the frequency of occurrence of BYDV outbreak leading to yield losses greater than the economic threshold justifying an insecticide spray (estimated to 0.5 t/ha). It reflects the production situation of a field.
The gap between CDR(Sopt) and CP (cost of a perfect decision rule) helps to estimate the financial interest achievable with improved indicators (Fig. 6). More accurate indicators will be more widely adopted by farmers because both their financial advantage and the range of production situations where they are of interest will be increased [78]. For example, in our case study, an improvement could be achieved by taking into account the proportion of migrant winged aphids that carry BYDV in autumn which was shown to vary according to land use at the regional scale and day-degree accumulation in spring and summer [79]. However, because of the inescapable trade-offs between the accuracy of decision rules and their user-friendly characteristics, it is important to keep in mind that even the most accurate decision rule will not be used if it requires too much effort from the user. Moreover, both uncertainty in modelling the “true” epidemiological process and uncertainty in the observation process limit the quality of indicators [78].
5 Plant resistance
Breeding cultivars resistant to aphids and/or viruses is an alternative control method particularly appealing in the context of sustainable agricultural development. Indeed, genetic resistance can confer an effective protection without additional costs or labour for farmers during the growing season. Moreover, genetic resistance is also safe for both the environment and human health. Thus, if resistance proves durable (i.e., still effective while used extensively for a long time in an environment conducive to the pest or disease – [80]), then the use of resistant crop varieties is clearly the preferred method to control agricultural losses.
Three mechanisms of resistance to aphids are described: (i) in antixenosis, the plant is rapidly recognised as a poor host by the pest that moves away; (ii) in antibiosis, survival and fecundity of the pest are affected by feeding on the plant; (iii) in tolerance, the plant is infested by pest that multiply, but is less affected than susceptible ones. The three types of resistance have been reported in a large number of aphid–crop interactions [81].
One of the oldest examples of the use of aphid resistant material for breeding, concerns the resistance of apple trees to the woolly apple aphid E. lanigerum. Some apple varieties were known since the middle of the 19th century as naturally resistant to this aphid in North America (Lindley, 1831, in Webster [81]): one of them, Northern Spy, has been extensively used during the first half of the 20th century as a parent in breeding programmes of resistant rootstocks at East Malling Research Station in the U.K. [82]. These rootstocks have been propagated all over the world and have been considered as aphid-resistant in most areas until now. However, the resistance could be locally broken by specific biotypes of E. lanigerum [83]. Another well-known example concerns the grape phylloxera, D. vitifoliae: this aphid attacking the root system of grape has devastated in the late 1800s the viticulture and the wine industry in many European countries. Grafting European susceptible ‘cepages’ on resistant American rootstocks (Vitis riparia, V. rupestris) has solved the problem [84].
In recent decades, considerable effort has been made in order to find and characterise sources of plant resistance to insects and/or viruses. Many attempts have been made to incorporate them into new cultivars, with a variable degree of success. At the end of the 20th century, more than 200 insect-resistant cultivars were grown around the world, among them around 25% resistant to aphids [85]. One impressive case concerns the ‘greenbug’ (Schizaphis graminum), a pest aphid of cereals in Northern America. At the end of the 1960s, a new biotype of aphid appeared, devastating sorghum crops. Resistant hybrids were selected and allowed a 90% reduction of insecticide applications in sorghum fields from 1972 onwards [82]. In the Netherlands, resistance genes of lettuce to the aphid Nasonovia ribisnigri have been characterised and the first resistant lettuce variety (Dynamite) was released in 1996, after 15 years of breeding [86]. Many varieties with this resistance source are now available and contribute to the control of aphid populations on this vegetable crop [87]. However, there are some recent reports on the occurrence of resistance-breaking N. ribisnigri biotypes in Germany [88]. In the apple, at least one resistant variety to the aphid D. plantaginea exists, Florina, but had no success because of conflicts with other agronomical, industrial or taste requirements [89].
No wheat or barley variety sufficiently resistant to the cereal aphids S. avenae, Metopolophium dirhodum and R. padi could be bred in Europe, in spite of intensive research [90]. No source of resistance was found in hexaploid wheats, but only in diploid ones and only against S. avenae [91]. The line Triticum monococcum REB81044 (TM44) was the most intensively studied. Life-history traits of S. avenae are dramatically affected by TM44, e.g., fecundity divided by 10 in comparison to a susceptible hexaploid cultivar [92,93]. In these experiments, the resistance was proven to be very specific, as other aphid species were not or little affected. Resistance affects S. avenae feeding and, interestingly, hinders virus transmission by this species only [94] (Fig. 7). Unfortunately, resistance genes from T. monococcum were difficult to incorporate into hexaploid wheats.
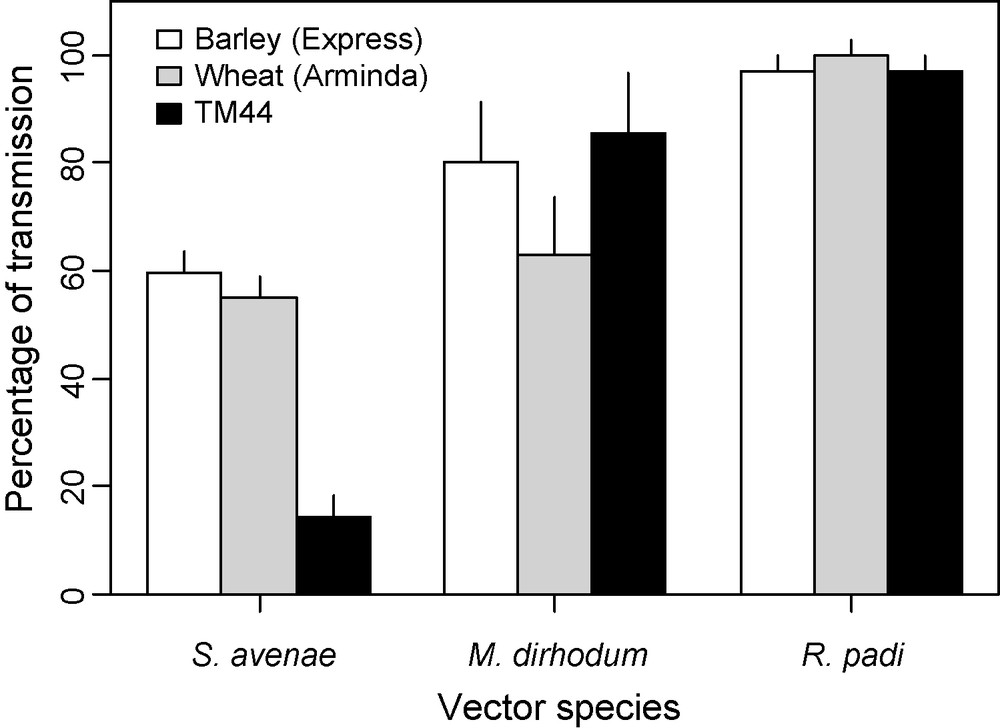
Percentages of transmission of BYDV-PAV to barley, hexaploid wheat and to the diploid wheat T. monococcum line 44 (resistant to S. avenae), by 3 aphid vector species [90].
Resistance genes have also been found against many viruses and, in several cases, cultivars with varying degrees of resistance are available to farmers. An updated list of published virus resistance genes is available in Kang et al. [95]. For further information on the mechanisms and durability of genetic resistances to plant viruses, the reader is referred to Lecoq et al. [96], Gomez et al. [97] and particularly to Dogimont et al. ([98], this volume) for a description of the single dominant Vat gene conferring melon resistance to Cucumber mosaic virus (CMV) transmission by Aphis gossypii.
6 Biological control and cultural management practices
Aphids are attacked by a large range of natural enemies. These include microorganisms, especially entomopathogenic fungi (Entomophthorales and Hyphomycetes), predators and parasitoids. Although their ability to regulate aphid populations in the long term is questionable [22], their short-term efficiency is beyond doubt. They have long been used as biological control agents with various degrees of success (for a review, see Powell and Pell, [99]). The three main strategies of biological control detailed below have been used against aphids. Moreover, several farming practices could allow avoiding or reducing crop infestation by aphids, and could be used alone or in association with biological control.
6.1 Inoculation
Inoculative or classical biological control concerns accidentally introduced pests. Exchanges of members of the old and the new worlds’ aphid fauna have been quite numerous since the early 20th century and have increased since the 1950s. In France for instance, 33 aphid species have been introduced from other countries between 1950 and 2005 [100,101], and among them 12 originated from North America. As an example, Illinoia liriodendri and I. morrisoni have been detected in the late 1990s and have spread in most of the country since their introduction [102]. The principle of classical biological control is to introduce one or more natural enemies originating from the same geographic area as the invasive species to control its populations. Such introductions have been done against many introduced aphids in numerous countries and mainly employed parasitoids. The first one was Aphelinus mali (Hymenoptera: Aphelinidae), which was successfully introduced in France and in many other countries around 1920 to control the woolly apple aphid, E. lanigerum, in apple orchards. Nowadays, this aphid is still under control in most apple production regions. However, A. mali is less efficient in western France, probably because of climatic factors [103]. The long time efficiency of successful inoculative biological control is one of its main advantages, together with the generally low initial technical and financial investment. For instance, only 225 individuals of the parasitoid Pauesia cedrobii were introduced from Morocco in 1981 in the south of France against the cedar aphid Cinara laportei, originating from the same country [104]. Twelve years later, the parasitoid has reached the area of Paris without any other specific intervention [105]. Conversely, some classical biological control programmes against aphids have been developed at a continental scale and have needed considerable resources. From the late 1980s to the mid 1990s, more than 15 million individuals of 10 parasitoid species and a few species of predator were introduced in North America to control the Russian wheat aphid, D. noxia [106]. Surprisingly, D. noxia seems nowadays to be regulated by native or by formerly introduced parasitoid species, rather than by the newly mass-introduced natural enemies.
Not only parasitoids could be good candidates for inoculative biological control programs. In Australia, several natural enemies were introduced around 1977 to control the alien aphid Therioaphis trifolii: the most efficient control agent was the fungus (Entomophthorale) Zoophthora radicans [107].
6.2 Augmentation
Augmentation is the second strategy of biological control, it concerns native pest insects. The aim of this method is to enhance the control of pests by rearing and releasing indigenous natural enemies. In the past, augmentation has been considered as a potential alternative to chemical control and research was conducted in the 1970s and 1980s on the various steps of mass production, insect conservation and release of aphid predators, entomopathogens and parasitoids. Despite these efforts, there are very few reports of successful augmentative releases of natural enemies against insect pests outdoors. Today, the use of augmentation for aphid control is limited to a few species attacking flower and vegetable crops in glasshouse, namely A. gossypii, M. euphorbiae, M. persicae, Aulacorthum solani and Macrosiphum rosae. Both predators (4 species) and parasitoid insects (3 species) are commercially available for growers and widely used especially in Europe. The parasitoids can be released directly or introduced in glasshouse by mean of banker plants. These plants are infested by an aphid species which is not harmful for the crop but allows parasitoid development. This ensures the presence of active parasitoids at the very beginning of the growth of the pest aphid populations. An entomopathogenic fungus, Lecanicillium longisporum (formerly Verticillium lecani) has been successfully mass-produced and used for limiting aphid populations in glasshouse. Because of its temperature and humidity requirements, it is only used in north European countries. The best results have been obtained in Chrysanthemum glasshouse because this plant has few fungal pathogens and the daily period of high humidity could be lengthened by sprinkling water and covering the plants with plastic [108]. The registration of ‘Vertalec®’, the blastospore preparation against aphids, is still pending in France.
In field situations, controlling aphid populations by mass release of natural enemies (inundation) is much more difficult. Recently, Levie et al. [109] have achieved control of cereal aphids, which were maintained below the economic threshold in experimental fields, by releasing thousands of the parasitoid Aphidius rhopalosiphi individuals. However, the authors evaluated the cost of the treatment as at least 20 times too high to have an economic interest. In fact, this approach seems more or less withdrawn for aphid control in fields and at the moment, the research efforts concern mainly the third biological strategy named conservation.
6.3 Conservation
Conservation aims at enhancing the population of the natural enemies present in the agro ecosystem, by means of habitat management and/or manipulation of their behaviour. As many different types of natural enemies attack aphids and could combine to maintain the pest population under the economic threshold, conservation biological control seems a promising way for limiting aphid damage and reducing pesticide use. In recent years, several works have been published on this topic. The limitation of pesticide use can be considered as the first step of conservation biological control, as avoiding insecticides could save insect predators and parasitoids and avoiding fungicides could save naturally efficient Entomophthorales [110]. In addition, the survey of natural enemies to determine whether they are able to control the aphid population without using insecticides is also generally considered as a conservation method. Moreover, it is possible to forecast the role of natural enemies in controlling populations. This has been especially developed with Entomophthorales because of the ability of some species, particularly Pandora neoaphidis (Fig. 8) and Neozygites fresenii, to cause natural epizootics reducing dramatically aphid populations within a short time [111]. This occurs during periods of high air moisture and mild temperature [112]. Mathematical models forecasting aphid dynamics under control of Entomophthorales have been developed [113,114], but are difficult to apply in the field. Their only practical application concerns the forecast of A. gossypii control by N. fresenii on cotton in the southern States of the USA. [107].
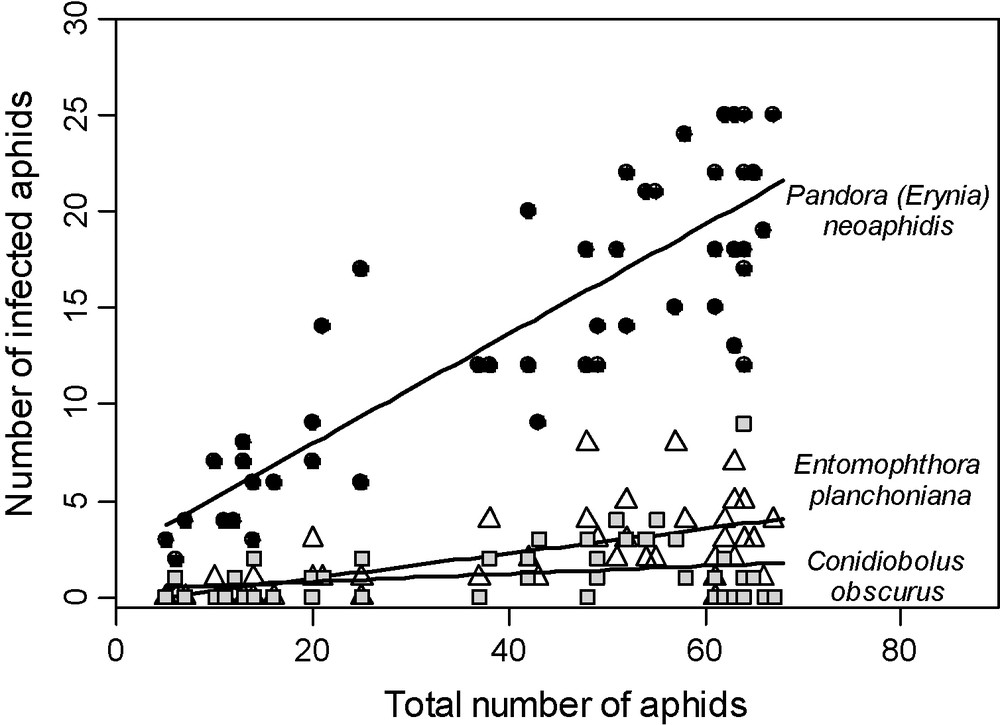
Two cases of density dependence of Entomophthorales species in S. avenae populations in wheat, in July 1980. X-axis: total number of aphids on one plant; Y-axis, number of aphids infected by one of the three Entomophthorales species.
Habitat management could be designed to provide food resources for natural enemies [115]. For instance, sowing flowering plants on the field margins could provide either pollen for adult insects whose larvae are aphid predators, like hoverflies [116], or nectar for adult parasitoids [117]. A positive impact of strips of Phacelia tanacetifolia along wheat fields on the populations of the cereal aphid S. avenae has been demonstrated [118].
Field environment could also be manipulated in order to provide: (i) alternative hosts for entomopathogens or parasitoids; or (ii) alternative prey for predators. In this case, the aim is to enhance the populations of these alternative hosts or prey, in order to favour indirect interactions between them and the aphid pest, especially apparent competition. Apparent competition between two herbivores occurs when they share a natural enemy, the presence of the first one increasing the predation or parasitism risk for the second one [119]. The natural occurrence of such an interaction has been demonstrated in aphids [120]. Recent studies report field experiments involving the establishment of plant strips bearing alternative hosts for parasitoids near cereal crops [109,121,122]. Results confirm that apparent competition could involve an enhancement of natural enemy impact, although it is difficult to ensure the efficiency of this method. In the same way, Entomophthorales attacking crop aphids could also develop on alternative aphid hosts feeding on non-cultivated plants, such as Microlophium carnosum on nettle (Urtica dioica), or S. avenae on Yorkshire fog (Holcus lanatus) [107]. However, experimental work should state that manipulated field margins or strips have no severe drawback for adjacent crops (e.g., as disease or pest reservoirs). A sound knowledge of the structure of aphid and their natural enemy communities is needed to develop such approaches (see also Le Ralec et al., in this volume [123]).
6.4 Cultural management practices
Some kinds of habitat management, like farming practices do not target natural enemies, but allow one to limit aphid populations or/and virus spread, generally by avoiding coincidence between aphids and susceptible stages of the crops. A good example is the late sowing of winter cereals (Fig. 9): R. padi populations migrating in autumn from maize to young cereals peak in September and the first two weeks of October, and later, migrant numbers decrease drastically. As a result, cereals sown at the beginning of October are in any case strongly contaminated, whereas those sown at the end of October escape 90% of the aphids [124]. Many other approaches are susceptible to reduce plant contamination by aphid or/and aphid multiplication on crops, like plastic mulches, kaolin particle films, fertilizer managements… For an exhaustive information on these methods, the reader is referred to Wratten et al. [125]. Finally, other methods can potentially reduce locally the number of aphid and virus reservoirs during the intercropping season, like ploughing volunteers of cereals and potatoes that are often heavily infested by aphids and infected by viruses [126].
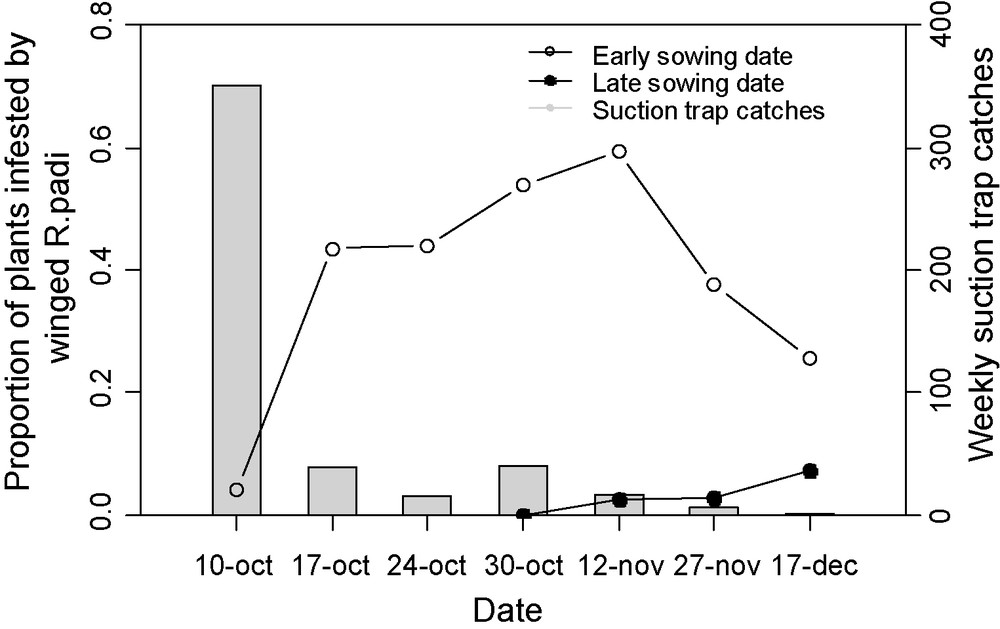
Effect of barley sowing date on autumn contamination by the aphid R. padi. In column the numbers of R. padi alates weekly caught by the suction trap in Le Rheu (Ille-et-Vilaine, France). In dotted lines the corresponding infestation of barley plots sown in le Rheu (i) at the beginning of October 2002 (early sowing), and (ii) at the end of October (late sowing).
7 Conclusion: Towards ecological aphid management strategies
During the last 20 years, fundamental research on aphids strongly increased and obtained exciting results of general scientific value (see this volume). At the same time, applied research targeted at reducing the damage that aphids inflict to crops also made significant progress. Examples include successes achieved in modelling aphid population dynamics and epidemiology of aphid-borne viruses, in providing routine molecular tools quickening the detection of insecticide resistance, and in monitoring at the European scale aphid flight with suction traps networks (the EXAMINE database, [127]). However, a large proportion of this new knowledge has still not reached farmers or advisers and, in practice, few novelties were introduced to improve aphid control strategies. Decision-making tools for insecticide management are still scarce, aphid-resistant varieties still scarcer and the real use of natural enemies restricted to some glasshouse crops. Maybe the most significant progress is the publication of bulletins monitoring (and sometimes forecasting) aphid population dynamics (e.g., the Aphid Alert Web Bulletin in Minnesota [128], the weekly bulletins from the Scottish Agricultural Science Agency, and many others). These bulletins aim to rationalize insecticide use and promote the adoption of “improved” rather than truly “integrated” pest management practises [129].
There is nowadays evidence that control strategies must be deployed at a scale matching the one at which pest and disease population dynamics naturally occurs [130]. There is also evidence that landscape complexity often favours natural enemy populations and decreases pest pressure [131] although differential responses of aphids to landscape complexity have been reported [131]. Landscape and regional factors are recognized to shape the epidemiology of aphid-borne viruses such as BYD viruses [23,132]. Accordingly, in our view, only aphid management strategies combining control methods acting at field, landscape and regional scales are able to go towards truly “Integrated” Pest Management. Reintegrating the field in this wider context is the only way to understand fully, and efficiently drive in a sustainable manner, the survival, multiplication and dispersion of aphids and of their enemies into the target field. This is achievable by combining individual and collective tools. Some of these tools, such as aphid trapping nets which are of great practical value, already exist [133] but must be maintained whereas the current tendency is progressive surrender. Others tools (e.g., safer insecticides, new varieties resistant to aphids and/or attracting natural enemies [134], new biological control strategies) have still to be developed from recent fundamental results such as aphid gene mapping, knowledge of aphid-symbiont physiology, elucidation of molecular mechanisms of virus transmission, polyphenism, switch between sexuality and parthenogenesis, and behavioural modelling of the relationships between aphids and their natural enemies.
Finally, one of the main obstacles to the adoption of innovative control strategies is that, if chemical control is rather cheap, simple to use and globally efficient, new strategies are often technically much more difficult to handle, more time-consuming and still subject to many hazards. Farmers logically tend to adopt cost-effective control strategies often minimizing their own immediate financial risk. The uncertainty associated with the impact of natural enemies on pest population drives many farmers to apply insecticides. However, filling this gap is challenging: it requires, for example, assessing the impact of natural enemy complexes not only on aphid populations, but also on yield increment, under different pest management strategies. Beyond the obvious need for research, the need for better cooperation among farmers, advisors and researchers will become more and more important as the degree of sophistication of management strategies increases [135]. Pedagogy must also be directed toward consumers who too often forget that asking for “clean, zero-fault” fruits and vegetables is a major obstacle to the renunciation of aggressive control measures by farmers.
Acknowledgements
Authors are especially grateful to Dr Caroline Petit-Lelaidier and two anonymous reviewers for improvement of the English of this manuscript.