1 Introduction
Heavy metal contamination has disastrous effects on plant productivity and threatens human and animal health. The presence of lead in the environment can cause serious problems to plants and animals. It has become a major environmental contaminant due to the rapid industrialization and urbanization. According to the environmental protection agency (EPA), lead (Pb) is the most common heavy metal contaminant in the environment [1]. Lead is not included in essential elements for plants, but they absorb it when it is present in their environment, especially in the rural areas where the soil is polluted by automotive exhausts and in fields contaminated with fertilizers containing heavy metal ingredients [2–4]. The effect of lead depends on the concentration, type of salt, soil properties and plant species [5]. Toxic levels of lead affect plant processes, as the metal reacts with important functional groups in macromolecules, and the activity of several enzymes is modified, some of which are important in photosynthesis, plant water status and mineral nutrition [6]. The major processes affected are seed germination, seedlings growth, tolerance index, dry mass of roots and shoots [7]. When lead enters the plant cells, like various heavy metals, it induces an oxidative stress in growing plant parts due to enhanced production of reactive oxygen species (ROS), and cell damages result in a reduction of plant productivity [8,9]. Plants have antioxidant systems to protect them against oxidative damage. Those detoxification processes are complex and highly compartmentalized in plant cells. The level of ROS in wheat seedlings is controlled by an antioxidative system that consists of antioxidative enzymes and non-enzymatic low molecular mass antioxidants. Superoxide dismutase (SOD, EC 1.15.1.1) is a key antioxidative enzyme that catalyzes disproportionation of superoxide anion (O2−) to H2O2 and O2. Catalase (CAT; EC 1.11.1.6) localized in peroxysomes, scavenges H2O2 by converting it to H2O and O2. Peroxidase (POD; EC 1.11.1.7) reduces H2O2 using several reductants, of phenolic compounds. POD is also the key enzyme in lignin biosynthesis participating in the formation of radicals of lignin units before their polymerization [10,11]. The two enzymes Ascorbate peroxidase (APX; EC 1.11.1.1) and glutathione S-transferase (GST; EC 2.5.1.18) play a pivotal role in scavenging ROS and maintaining the level of antioxidants ascorbate and glutathione [9]. In addition, GST is involved in the detoxification (conjugation) of lipid peroxidation products (unsaturated alkenals) [12].
Seed germination is the initial event in the life of a plant and is initiated with regulation of enzymatic reactions which activate catabolic and anabolic processes in the storage tissues and the embryonic axis, respectively. Germination is inhibited even if a single component of these processes is affected. One recognized explanation of the impact of heavy metals on the plant physiology is that it results in several nutritional disturbances. Breakdown of storage compounds is among the crucial events that govern seed germination following water imbibition [13,14].
The effects of heavy metals in this process, and the protection of embryos are not well documented. To our knowledge, a few works only have been performed on the mechanism of lead phytotoxic effects on the process of germination on agricultural crops. In particular, the degree to which exogenous lead is able to pass through the seed coat into the seed and consequently affect the process of germination remains an open question [15,16].
The purpose of the present study is to contribute to the understanding of the biochemical changes in germinating seeds subjected to heavy metal stress. We present in this work the extent of lead uptake by wheat seedlings and its impact on germination, by analyzing its morphological and biochemical effects during the first 6 days of germination of Triticum aestivum seeds.
2 Material and methods
2.1 Growth and seeds treatment
Effects of different concentrations of Pb(NO3)2 (0, 0.05, 0.1, 0.5, 1 g/L, corresponding to 0, 0.15, 0.3, 1.5 and 3 mM of lead) on germination, biomass and plantlet shoot and root length were evaluated. The lead solutions were freshly prepared by dissolving Pb(NO3)2 in deionized water and adjusting their pH to 5.5 with HNO3.
A variety of wheat (Triticum aestivum L. cv. Achtar) was provided by the National Institute of Agronomical Research (INRA), Tangier, Morocco. Prior to germination, seeds were surface-sterilized with 5% (v/v) sodium hypochlorite for 10 minutes and rinsed several times with distilled water. Seed germination was tested on filter papers placed in Petri dishes and moistened with 6 ml aqueous lead solution. Controls were obtained by moistening the filter papers with 6 ml deionized water. Thirty seeds of each variety were placed in each dish and incubated in the dark at 25 ± 1 °C and the proportion of seeds that had germinated after 3 days was counted. Seeds were considered to have germinated when both the plumule and radicle were over 2 mm long. Seedling biomass was also determined and each treatment was set up in triplicate.
2.2 Determination of lead concentration and growth analyses
After 6 days’ growth, five uniform seedlings were selected from each pot for the determination of lead concentrations. Seedlings were first rinsed four times with deionized water. They were then oven dried at 70 °C for 48 hours, weighed and digested with ultra-pure concentrated HNO3 (5 ml) and 30% w/v H2O2 (3 ml). Lead was determined by atomic absorption spectroscopy (VARIAN, AA 240 FS). All measurements were performed in five replicates.
The elongation of roots and leaves was measured with a ruler. Calculation was performed on the base of the length of leaves and the average of major axial roots and collected from at least 20 independent plants on each Petri dish. For the determination of biomass (fresh weight), tissue samples were blotted on Whatman paper and weighed.
2.3 Soluble protein and proline determination
Soluble protein were quantified according to Bradford [17]. Samples (leafs and roots) were homogenized in 0.1 M Na-phosphate buffer (pH 7). Absorbance was recorded at 595 nm and concentration was calculated using bovine serum albumin as standard.
Proline content was measured according to the method described by Bates et al. [18]. Fresh seedlings (0.5 g) were ground in 3% (w/v) aqueous sulphosalicylic acid and proline was estimated by ninhydrin reagent. The absorbance of the fraction with toluene aspired from the liquid phase was read at 520 nm. The proline concentration was determined after the realization of a standard curve; it was expressed in μmol/g fresh weight.
2.4 α-Amylase activity and Esterase determination
Amylolytic activity (α-Amylase: EC 3.2.1.1) was measured according to the method of Valencia et al. [19], using iodine. Seeds were homogenized in citrate buffer 10 mM Na-NaCl-CaCl2 at pH 8. The mixture was added to 0.5% (w/v) starch solution and incubated at 37 °C during 15 minutes. 2.5 ml of iodine reagent (I2; KI) was added and centrifuged at 4000 g for 10 minutes. The absorbance was read at 580 nm. The activity of the enzyme was expressed as μg of consumed starch/min/mg protein.
Esterase activity (EST; EC 3.1.1.1) was evaluated by the method of Karoly et al. [20], with some modifications. The activity was assayed for 15 minutes in a reaction solution composed of 1 ml of 0.6 mM β-naphthyl acetate in 0.1 M Na-phosphate buffer (pH 7) and 1 ml of supernatant and incubated at 30 °C; the reaction was quenched by adding 240 μL of a dye solution prepared by mixing fast garnet with SDS. Naphthol-fast blue complex was formed and measured after 15 minutes at 492 nm; β-naphthol was used as standard.
2.5 Determination of lipid peroxidation and antioxidative enzymes
Wheat leaves and roots (100 and 50 mg, respectively) were separately homogenized in 1 mL ice-cold extraction buffer containing 0,1 M Na-phosphate buffer (pH 7), 1 mM EDTA, 1 mM PMSF and 0.5% (w/v) of PVP. The homogenate was centrifuged at 9000 g for 20 minutes. The supernatant was used as the crude extract for determination of lipid peroxidation and assay of enzyme activities.
Accumulation of lipid peroxides in tissues was determined in terms of thiobarbituric acid reactive substances (TBARS), by estimation of malondialdehyde (MDA) content based on the method of Health and Packer [21]. Supernatant (100 μl) was mixed with 1 ml of 0.1% (w/v) trichloroacetic acid (TCA) solution. The homogenate was centrifuged at 10,000 g for 5 minutes and 200 μl of the supernatant was mixed with 0.8 ml of 0.5% (w/v) thiobarbituric acid (TBA) in 20% TCA. The mixture was heated at 95 °C for 30 minutes, chilled on ice, and centrifuged at 10,000 g for 5 minutes. The absorbance of the supernatant was measured at 532 nm. The value for non specific absorbance at 600 nm was subtracted. The amount of TBARS was calculated by using the extinction coefficient of 155/mM/cm.
Catalase activity was measured as described in Beers and Sizer [22]. The activity was assayed with addition of 500 μl of supernatant to 500 μL of 1% H2O2 solution. The mixture was incubated in 37 °C during 10 minutes. 500 μl of KI (10%) was added thereafter and the absorbance read at 470 nm. The enzymatic activity was expressed in μmoles of consumed H2O2/min/mg of protein.
Guaiacol peroxidase (POD, EC 1.11.1.7) activity was measured following the change of absorbance at 470 nm due to guaiacol oxidation. The activity was assayed for 3 minutes in a reaction solution (1 ml final volume) composed of 100 mM Na-phosphate buffer (pH 7.0), 0.6 mM guaiacol, 10 mM H2O2 and 50 μl of crude extract, as described in Putter and Bergmeyer [23].
Superoxide dismutase (SOD, EC 1.15.1.1) was assayed by measuring its capacity of reduction of nitro-blue tetrazolium (NBT) by xanthine oxidase-generated superoxide [24]. The reaction mixture (1 ml) contained 50 mM Na-phosphate buffer (pH 7.8), 10 mM methionine, 1.17 mM riboflavin, 2.24 mM NBT, 1.33 mM diethylene triamine pentaacetic acid (DETAPAC) 1.8 mM xanthine, 50 μl of xanthine oxidase (0.25 U/mL) and 20 μl enzyme extract. The absorbance of solution was measured at 560 nm. One unit of SOD was defined as the enzyme activity that inhibited the reduction of nitroblue tetrazolium to blue formazan by 50%.
Total GST (GST; EC 2.5.1.18) activity was measured by the method of Habig et al. [25], using 1-chloro-2,4-dinitrobenzene (CDNB) as a substrate. The reaction mixture contained 0.1 M potassium phosphate buffer (pH 6.25), 0.75 mM CDNB, 30 mM GSH and enzyme extract. The increase in absorbance due to the formation of the conjugate (ɛ = 9,600 L/mM/cm) between GSH and CDNB was monitored at 340 nm. Enzyme activity was expressed in units, each representing 1 μmol (S-conjugates formed)/min.
Ascorbate peroxidase (APX) activity was measured by the decrease of ascorbate absorbance at 290 nm. The reaction mixture contained 50 mM of Hepes buffer (pH 7.6), 0.25 mM ascorbate and 0.1 mM H2O2 [26].
2.6 Statistics
Data were subjected to one-way analysis of variance (ANOVA) using Statistica Software. Post-hoc testing was carried out using the Tukey test. A significant level of 0.05 was used for all statistical tests.
3 Results
3.1 Lead accumulation and plant growth
Fig. 1 shows the accumulation of lead in wheat seedlings after they were treated with different concentrations of lead for 6 days. Our results show that the accumulation of lead increases linearly with increasing lead levels in the medium. Seedlings grown under 3 mM lead for 6 days showed up 1.08 mg/g dry weight of absorbed lead in the seedlings, and the difference with controls for 1.5 and 3 mM was highly significant (P <0.001).
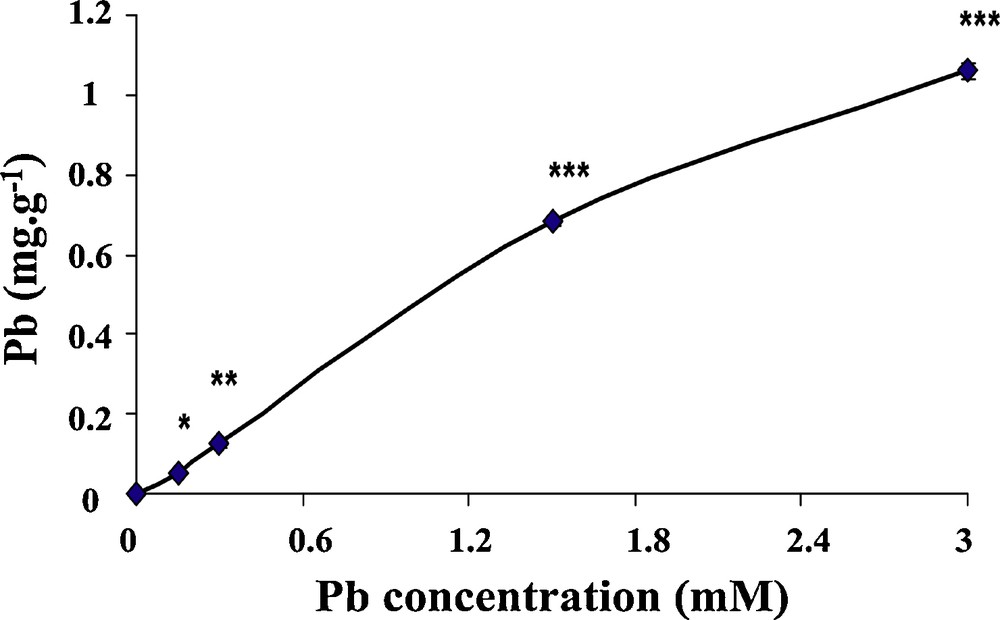
Lead residues in wheat seedlings. Wheat seeds were germinated in aqueous solutions containing lead (0–3 mM) for 6 days. After treatment, seedlings were harvested and the content of lead was determined as described in M&M. Vertical bars represent standard deviation of the mean (n = 10). Asterisks indicate significant differences of the lead contents in wheat seedlings (*p < 0.05; **p < 0.01; ***p < 0.001).
Visible symptoms were clearly demonstrated by the morphological parameters of growth (Fig. 2). In controls, percentage of germinated seeds reached 98%, whereas it was only 71% in the treated seeds at the concentration of 3 mM (Fig. 2A). The percentage of germination decreased in a dose-dependent manner. The reference weight for seeds before imbibition is 45 mg. Control seeds weight doubled during the treatment period (92.8 mg). On the other hand, at lead concentrations of 1.5 and 3 mM, the weight reached only 75.9 mg and 52.2 mg, respectively (Fig. 2B). The difference is statistically significant (P <0.05) between the weight of controls and that of plants treated with 1.5 and 3 mM. After 6 days, wheat seed was already ruptured and visible protrusion of radicle indicated the onset of embryonic axis elongation. This lengthening increased during the period of germination (Fig. 2C). After lead treatment at 3 mM, lengthening was much reduced; it reached only 2.3 cm on the 6th day, whereas in the control, it reached 7.7 cm. The difference in root length is high significant (P <0.001) between the controls and the seeds treated with 3 mM. The length of control roots reached 9.64 cm in the 6th day.
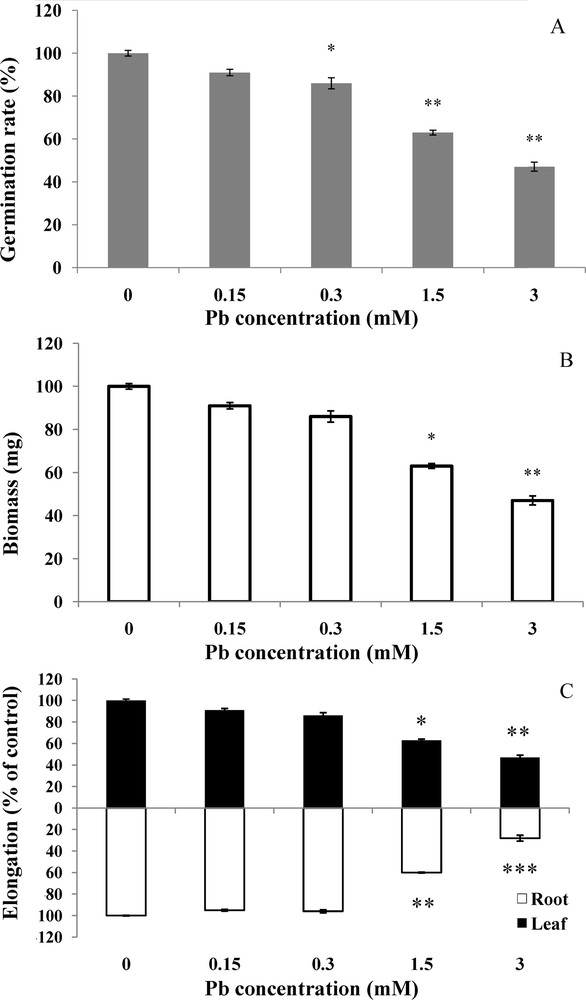
Effect of lead on wheat germination and growth. Wheat seeds were germinated in aqueous solutions containing lead (0–3 mM) for 6 days. Then, the germination rate (A) biomass (B), and elongation of both roots and leaves (C) were measured, respectively. Values are the means ± SD (n = 5). Asterisks indicate significant differences between the treatments and the control (*p < 0.05; **p < 0.01; ***p < 0.001).
3.2 Effect of lead on soluble protein and proline accumulation
To examine the biochemical responses to lead, we first determined the effect of lead on soluble protein content in wheat seedlings after 6-day treatment. As a result to the exposure to lead, soluble protein content showed a significant increase (P <0.05) trend with the increase of the concentration of lead (Fig. 3A). Soluble protein increased by 7.5, 11.1, 18.7 and 21% in roots, 19, 23, 28 and 34% in coleoptiles, at 0, 0.15, 0.3, 1.5 and 3 mM of lead, respectively.
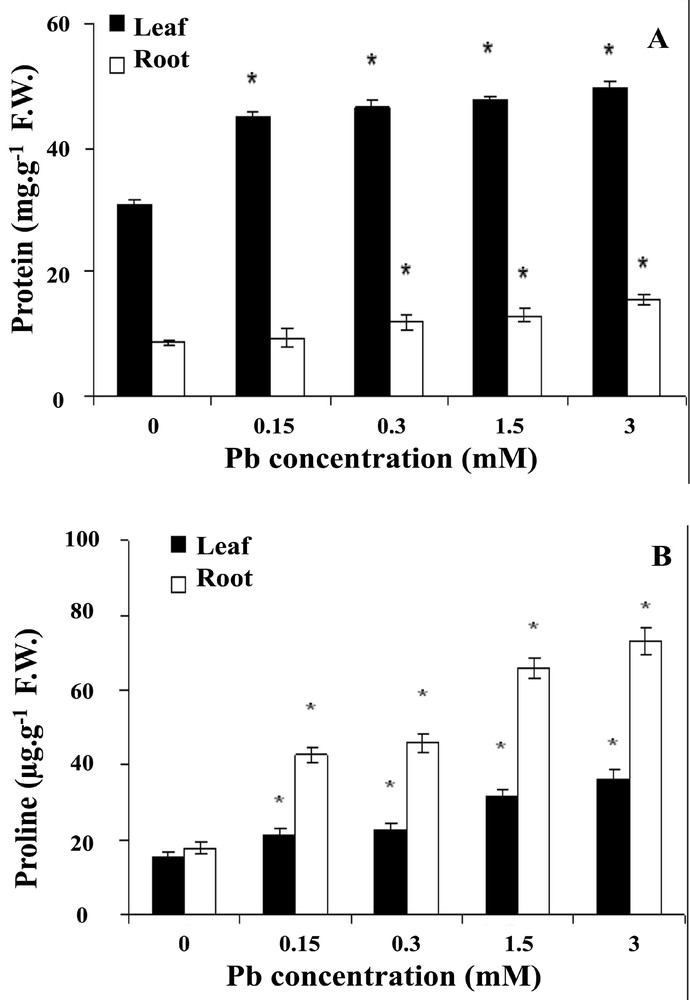
Effect of lead on soluble proteins (A) and proline (B) content in wheat seedlings. Wheat seeds were germinated in aqueous solutions containing lead (0–3 mM) between the treatments and the control (*p < 0.05).
Proline content of the roots was greatly enhanced with lead at all tested concentration; by contrast, the leaves appeared less sensitive to lead exposure (Fig. 3B). The increase of proline concentration in the roots is dose-dependent, and it increases by 366 and 411% in the roots treated with 1.5 and 3 mM of lead, respectively, compared to the controls at day 6.
3.3 Effect of lead on α-amylase and total esterase activities
Lead stress can induce catabolic metabolism disorders. In this study, the α-amylase activity in treated seeds showed a reduction of its activity as compared to controls (Fig. 4A). With increasing levels of lead, a concomitant decline in α-amylase activity was observed. Seeds growing under 1.5 and 3 mM lead showed about 38 and 72% decline in α-amylase activity, and statistical tests showed a significant difference in α-amylase activity between seeds grown in presence of lead and control seeds (P <0.05).
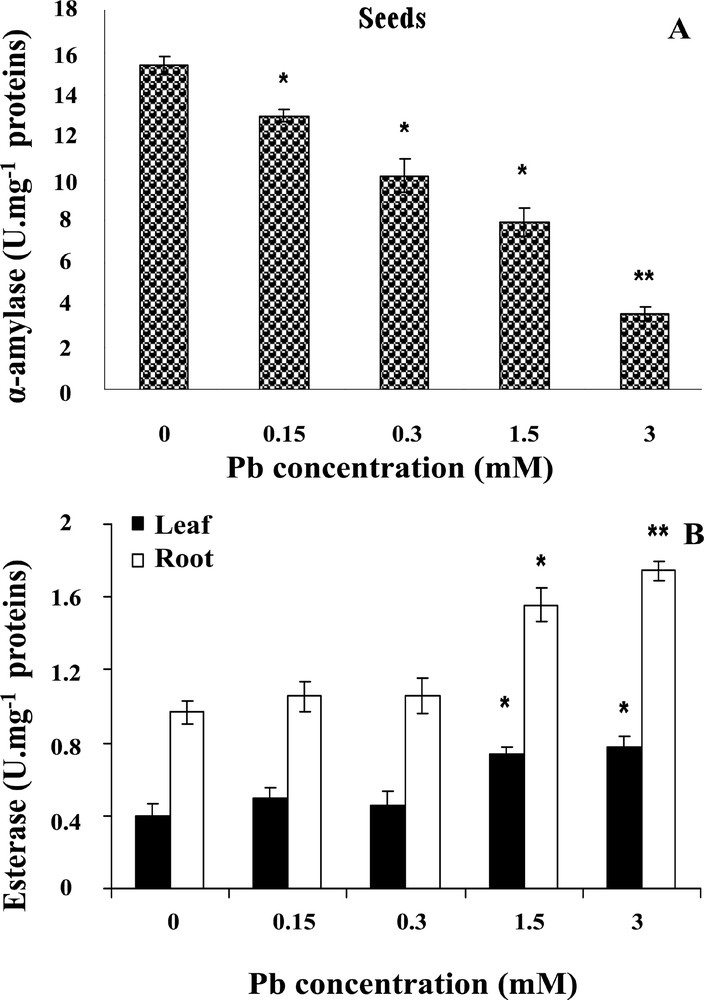
Effect of lead on α-amylase (A) and esterase (B) activities in wheat seedlings respectively. Wheat seeds were germinated in aqueous solutions containing lead (0–3 mM) for 6 days. Then, α-amylase and esterase activities were measured. Values are the means ± SD (n = 5). Asterisks indicate significant differences between the treatments and the control (*p < 0.05; **p < 0.01).
In control wheat seedlings, the activity of esterases was higher in roots than in coleoptiles. Esterase activity was increased in the presence of lead (Fig. 4B), and under lead treatment roots maintained a higher esterase activity than shoots; this stimulation was statistically significant (P < 0.05) in the presence of all concentrations used, and seedlings growing with 1.5 and 3 mM lead showed increases of about 66 and 114% in roots, and 72 and 86% in coleoptiles, respectively.
3.4 Effect of lead on lipid peroxidation and antioxidant enzymes activities
Exposure of wheat seedlings to lead induced lipid peroxidation in roots and leaves. The level of lipid peroxides, expressed as thiobarbituric acid reactive substances (TBARS). MDA is the product of membrane lipid peroxidation, and its content reflects the degree of cell membrane damage when exposed to ROS. Fig. 5 shows that MDA content increased slightly when the concentration was lower than 0.3 mM, but increased dramatically at high lead concentrations (1.5 and 3 mM). When lead concentration reached 3 mM, MDA content became 7- and 5-fold higher than in control coleoptiles and roots, respectively. The maximum of MDA accumulation is observed at 3 mM of lead, and the difference with controls is highly significant (P < 0.01).

Effect of lead on lipid peroxidation (TBARS) in wheat seedlings respectively. Wheat seeds were germinated in aqueous solutions containing lead (0–3 mM) for 6 days. Then, thiobarbituric acid reactive substances (TBARS) were measured. Values are the means ± SD (n = 5). Asterisks indicate significant differences between the treatments and the control (*p < 0.05; **p < 0.01).
Lead-induced oxidative stress was further confirmed by the dose-dependent increase of antioxidant enzyme activities. It can be seen in Fig. 6A that SOD activity increased under lead treatment. When the concentration of lead was 1.5 or 3 mM, SOD activity increased by 21 or 36%, respectively in roots, and this stimulation was statistically significant (P < 0.05) in both parts of seedlings.
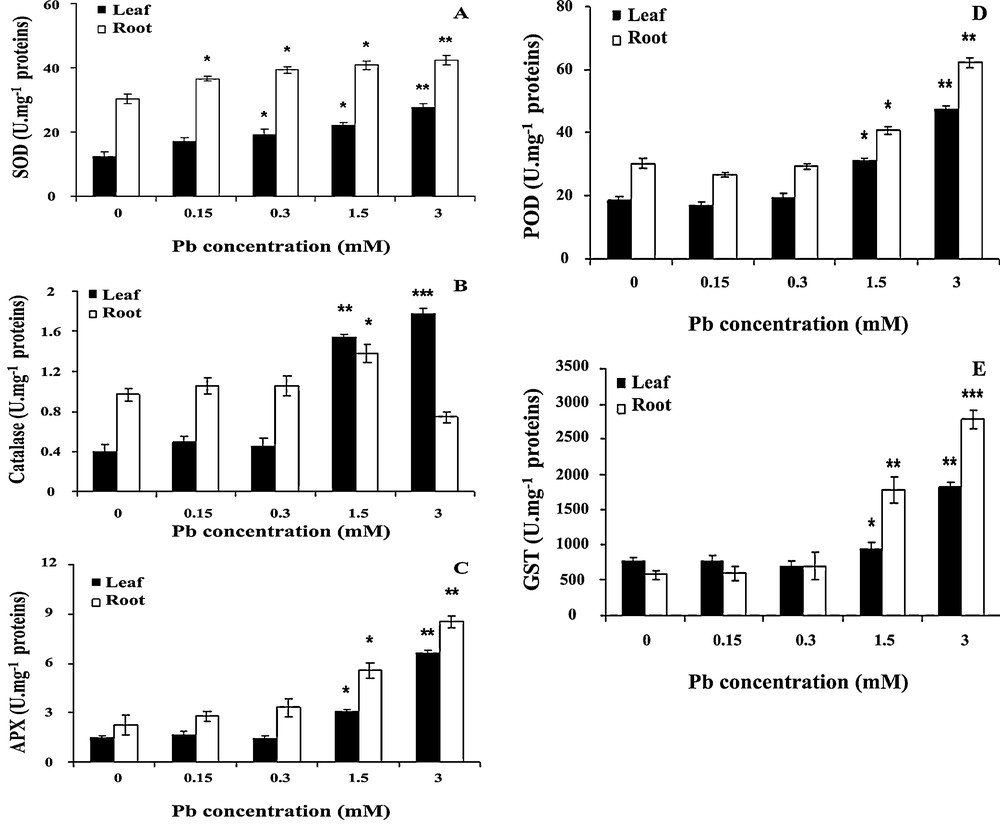
Effect of lead on the activities of superoxide dismutase (SOD) (A), catalase (CAT) (B), ascorbate peroxidase (APX) (C), peroxidase (POD) (D) and Glutathione S-transferase (GST) (E) in wheat seedlings. Wheat seeds were germinated in aqueous solutions containing lead (0–3 mM) for 6 days. Then, the enzyme activities were measured. Values are the means ± SD (n = 5). Asterisks indicate that mean values are significantly different between the treatments and the control (*p < 0.05; **p < 0.01; ***p < 0.001).
Effects of lead on CAT activity are presented in Fig. 6B CAT activity displayed increasing trends at higher concentrations in coleoptiles, and then decreasing trends with increasing concentrations of lead (3 mM) in roots. When the concentration of lead was 3 mM, CAT activity increased to 210 and 280% in coleoptiles at 0.5 g/l and 1 g/l respectively. However, it was reduced to 15% at the highest concentration of lead in roots. The differences in coleoptiles formed under 1.5 and 3 mM of lead are highly statistically significant (P < 0.01).
The effects of lead on APX and POD activities are presented in Fig. 6C and D, APX and POD activities increased significantly with the increase of lead concentrations. Up to lead concentrations of 0.3 mM, APX and POD activities remained similar to those of controls. APX and POD activities increased by 50% and more than 100% at lead concentrations of 1.5 and 3 mM, respectively (P < 0.05).
Activities of GST in roots and coleoptiles showed a similar pattern (Fig. 6E). Treatments with 1.5–3 mM of lead induced a progressive increase in activity; generally, the activity in roots was higher than this in the coleoptiles. GST activity increased by 150 and 280% in coleoptiles and roots, respectively at the highest lead concentration. This stimulation is statistically highly significant (P < 0.01) in both parts of seedlings.
4 Discussion
Lead is one of the most abundant and ubiquitously distributed toxic elements. It exerts adverse effects on morphology, growth and photosynthetic processes of plants and causes inhibition of enzyme activities, water imbalance, alterations in membrane permeability and disturbs mineral nutrition [6,27].
The present study on lead uptake showed an increased uptake of lead in wheat seedlings as lead concentrations increased in the growth medium. Lead is transported from the medium to the root cells by plasma membrane cation channels, especially the Ca2+-channels [28]. The present results demonstrate that in the lead contaminated solutions, wheat seedlings accumulate lead up to toxic levels. Wheat seeds are permeable to lead during the first stage of germination when water uptake is intense. In the end of this stage, when water uptake is reduced, seedlings become more permeable to lead. The lead that penetrated into the embryo in the final stage of imbibition delays germination [16]. It has been shown that high concentrations of lead caused a decrease of germination in rice seedlings and reduces their growth [29]. A considerable decrease in biomass in response to lead stress appears similar was reported in the responses of wheat to increase of lead in soil [15,29,30]. However, unlike our study, in corn seedlings, an apparent increase in plant organs was reported which was due to an increase in the synthesis of cell wall polysaccharides resulting from lead exposure [31]. The elongation of roots is very sensitive to the effect of lead. This parameter is more sensitive than the elongation of the coleoptile, because the root cells are in direct contact with lead. This inhibition reached more than 60% for the treatment with 1.5 mM lead. Several workers have reported the inhibition of root growth and of cell divisions in root tips, with mitotic abnormalities, damages to microtubules and destabilization of the cellular membranes [28].
Lead toxicity induces an increase of protein content in exposed wheat seedlings, possibly due to the induction of stress proteins under metal exposure [29]. These stress proteins may comprise various antioxidant enzymes and other enzymes involved in GSH and PC biosynthesis, and also some heat shock proteins [32]. Moreover, Rauser [33] indicated that this rise in the protein concentration can be also explained by the production of phytochelatins aimed to detoxify the high lead concentrations.
Proline concentrations increase with those of lead in the growth medium, and this increase is more relevant in roots than in coleoptiles [15].
Heavy metal treatments result in modifications of the activity of a wide range of enzymes. Some (e.g. α-amylase) show a reduced activity, whereas the activity of other ones (esterases) is enhanced. Thus, lead has been shown to considerably decrease the activity of hydrolytic enzymes, proteases and α-amylase [34]. Mihoub et al. [35] showed that the activity of α-amylase was inhibited in pea (Pisum sativum L.) seedlings treated with Cd or Cu ions. α-Amylase is active during germination and allows the mobilization of starch reserves, an essential process for providing substrates to growing cells, and its inhibition might explain the inhibition of germination/growth. It is possible that this inhibition results from a direct effect of lead ions on the enzyme, by displacing the Ca2+ ions that are essential for enzyme activity [36]. Esterases are ubiquitous enzymes present during all developmental stages, and esterase activity can be used as a marker of cell growth and lead toxicity [37]. Mukherjee et al. [38] tested esterase activity in exposed and unexposed Lemna minor (duckweed) to six heavy metals, and they showed that the specific activity of esterase increased in exposed populations. Thus, the increased esterase activity in wheat seedlings results from a stress reaction induced by lead exposure.
It is established that lead toxicity results in enhanced ROS generation [9]. The increase of TBARS formation is a direct consequence of increased ROS formation and thus of unsaturated fatty acid peroxidation. The levels of ROS in wheat seedlings are controlled as usual by a complex antioxidant system that consists of enzymes – catalase (CAT), peroxidase (POD), superoxide dismutase (SOD), ascorbate peroxidase (APX) together with scavenger enzymes such as glutathione S-transferase (GST) – and non enzymatic low molecular mass antioxidants such as glutathione (GSH), ascorbate, tocopherols carotenoids and proline. Proline belongs to this non-specific defense system against lead toxicity, as an inhibitor of lipid peroxidation [39], a free radical scavenger [40], as well as a metal chelator [41], and the increase of its concentration can be considered as a defense reaction against lead administration.
The current results show an increase of SOD activity in wheat seedlings growing in the presence of lead. SOD is considered as a first defense against ROS as it acts on superoxide radicals, which are produced in different compartments of the cell and are precursors of the other ROS [42]. Increase in SOD activity is attributed to the increase in superoxide radical concentrations. This is likely due to de novo synthesis of enzyme protein [9], which is attributed to transcription of SOD genes by a superoxide-mediated transduction signal [43].
Catalase activity increases under lead phytotoxicity, and this increase can be also explained by a substrate induction, in order to maintain low levels of H2O2 as an adaptive mechanism [44].
POD is located in cytosol, cell walls, vacuoles and extracellular spaces. It is considered as stress marker enzyme having a broad specificity for phenolic substrates and a higher affinity for H2O2 than CAT. POD consumes H2O2 to generate phenoxy compounds that are polymerized to produce cell wall components such as lignans [44]. Increase in POD is correlated with lead stress suggesting it to be an intrinsic defense tool [9]. Increased POD activity shown in our results can be related with the release of peroxidase localized in the cell walls [45].
Enzymes of ascorbate–glutathione cycle are localized mainly in chloroplasts and also in other cellular organelles and cytoplasm, where they play important role in combating oxidative stress. In the present study, APX activity was increased to very high levels at various concentrations with significant decrease only at highest concentrations and duration. Increased activity of APX may efficiently scavenge H2O2 to protect against oxidative damage. SOD, APX and GPX, in general, show simultaneous induction and decline, which may be due to their coregulation [46,47].
Glutathione-S-transferase catalyzes the conjugation of endogenous (or exogenous) electrophilic substrates to reduced glutathione (GSH). The resulting conjugates are transported to vacuoles for further processing or degradation [48]. Therefore, GST plays an important role in the removal of toxic products of lipid and protein peroxidation. It has been reported that GST may be involved in the resistance of plants to diverse environmental stresses [49]. In a similar way, an increase of GST activity was observed after treatment of wheat shoots with 200 μM Ni [50].
5 Conclusion
In conclusion, the present work has shown that lead exposure to wheat seedling induces a lot of metabolic disturbances, which result in a dose-dependent inhibition of germination or seedling growth. These effects are more marked in the roots, which are likely the organs exposed to the highest lead concentrations. This system with its different biomarkers will be used in future experiments aimed to search for molecules able to provide a protection against the deleterious effect of lead.
Conflicts of interest statement
No such conflict exists.
Acknowledgements
The authors are thankful to Pr. Mustapha Semmar (Educational inspector of English at the Academy of Tangier-Tetouan, Morocco) for his language improvement of the manuscript, Dr. Driss El Guerrouje, research professor in the Institut national de recherche agronomique (INRA) in Tangier, Morocco, for the use of the atomic absorption spectrophotometer.