1 Introduction
Vesicomyid bivalves are one of the most abundant chemosynthetic faunal species inhabiting deep-sea reducing ecosystems, including hydrothermal vents, whale falls and cold-seep ecosystems [1–3]. About 100 vesicomyid species are described worldwide mainly at bathyal and abyssal depths [4,5], and colonise the great majority of cold-seep sites. Vesicomyid bivalves live in symbiosis with sulphide-oxidising bacteria located in their gills [6,7]. These symbionts produce organic compounds used by bivalves for their nutrition and, in turn, the bivalve hosts supply their symbionts with oxygen, carbon dioxide and sulphide. Oxygen and carbon dioxide are directly available in the ambient seawater and are transported through the inhalant siphon to gills and the bacteria therein. Sulphide comes from the sediment and goes through the foot into the haemolymph and is thus transported to the gill [8]. Their metabolism is indirectly based on methane anaerobic oxidation (AOM) [9] producing sulphide used as energy source by the vesicomyid symbionts.
Metabolic rates of vesicomyids are not well known. Three studies on oxygen consumption rates have been performed ex situ measurements on hydrothermal vent vesicomyids in stainless steel vessels to maintain in situ pressure conditions during the experiment [8,10,11]. Arp et al. [8] assessed oxygen consumption rates in Calyptogena magnifica Boss and Turner, 1980 [12], and observed values were as high as those from shallow-water bivalves. Two other smaller species, Calyptogena elongata Dall, 1916 [13] and Calyptogena pacifica Dall, 1891 [14], show much lower oxygen consumption rates (average 0.2 μmol.g wet weight [without shell]−1h−1 [11]). Only one in situ measurement of oxygen consumption rate has been performed on C. magnifica on the Galapagos rift, giving an average rate of 0.4 μmol.g wet weight−1.h−1 [11].
The first in situ measurements of methane, oxygen and carbon fluxes were carried out in several deep-sea environments using benthic chambers [15–18]. However, these devices deployed from the surface cannot specifically target selected biogenic habitats in highly heterogeneous environments such as cold-seeps or hydrothermal vents. A relatively new approach is to deploy benthic chambers with submersibles. These devices have been used at seeps to measure both methane and oxygen fluxes, to assess the role of microbial activity, especially anaerobic oxidation of methane [19,20], and the role of macrofauna, particularly through symbioses [20–22] in the regulation of seabed methane emissions (benthic biological filters). Differences in total oxygen uptake (TOU) have been observed between active seeps and “normal” sediments (from 2 to 20 times higher in active seeps) [20,21] and among seep habitats [20,22,23], revealing higher fluxes in macrofauna clusters than in microbial mats on Håkon Mosby mud volcano (HMMV) [22]. In these papers, TOUs have been measured in situ by autonomous or ROV-manipulated chambers in microbial mats, vesicomyid beds, siboglinid or ampharetid polychaete fields. Only one in situ oxygen uptake estimation on vesicomyid bivalves has been obtained with a benthic chamber deployed from the surface, remotely guided for habitat selection, but without estimation of the density and biomass of individuals [20]. TOUs also vary according to advective methane fluxes and show a negative relationship across the HMMV ecosystem [22].
The giant Regab pockmark off Gabon, located at 3160 m depth along the Congo-Angola margin, is characterised by dense assemblages of vesicomyids, mytilids and escarpids distributed along a gradient of methane concentrations decreasing from the centre to the periphery [24]. Two species of vesicomyids – Christineconcha regab Cosel & Olu, 2009 [4,25] and Laubiericoncha chuni Cosel & Olu, 2008 – are found in the pockmark, where C. regab largely dominates. The giant pockmark was re-visited by the ROV Quest 4000 (MARUM, University Bremen, Germany) in 2008. We selected several vesicomyid beds in the centre and at the south-west border of the active area to: (1) perform in situ benthic fluxes measurements to estimate vesicomyid respiratory rates; and (2) compare TOU on clam beds of various density with different methane levels along this cold-seep ecosystem.
2 Materials and methods
2.1 Study site
The giant pockmark Regab (800 m wide and 15 m deep) was discovered in 2001 at 3160 m depth along the Congo-Angola margin [24]. Dense assemblages of symbiont-bearing species including Bathymodioinae, Escarpiidae and Vesicomyidae have been observed along a NE-SW axis [26]. Their distribution is consistent with the methane concentration in water above the sediment which is higher in the centre of the pockmark, where mytilids and siboglinids dominate, than in the periphery dominated by clam beds. Previous studies had enabled the sampling of C. regab all along the pockmark while L. chuni only in the periphery [4].
During the GUINECO expedition M76-3b aboard the RV Meteor (Chief scientist: A. Boetius), the Regab pockmark was re-explored with the ROV Quest 4000 (MARUM, University Bremen, Germany). Following previous studies, two sites were studied along the NE-SW axis: (1) at the centre (Site 1, Marker GUINECO 7) where the highest methane levels in vesicomyid beds were recorded in 2001 (3.9–4.4 μM); and (2) to the south-west (Site 2, Marker GUINECO 10) where methane concentrations were roughly four times lower (0.9–1.0 μM) (Table 1, Fig. 1). At each site, water temperature below the sea-floor recorded by the ROV Quest 4000 was 2.6 °C. As previously observed [4], these sites were characterised by dense aggregates of vesicomyid bivalves. During the GUINECO cruise, C. regab being sampled at both sites, whereas a few individuals of L. chuni was also sampled in the SW vesicomyid bed.
Study sites at the REGAB pockmark, number of individuals sampled and number of individuals counted under CALMAR with photos for C. regab (Cr) and L. chuni (Lc).
Site (Marker GUINECO) | Latitude | Longitude | Depth (m) | Dive number (Pangaea labels) | No. of individuals sampled | No. of individuals under CALMAR |
Centre (M7) | S 5° 47.8674 | E 9° 42.6881 | 3171 | M76/3b 355, D220 | 80 Cr | 46 Cr |
South-West (M10) | S 5° 47.9761 | E 9° 42.4825 | 3170 | M76/3b 379, D225 | 143 Cr + 8 Lc | 121 Cr + 4 Lc |
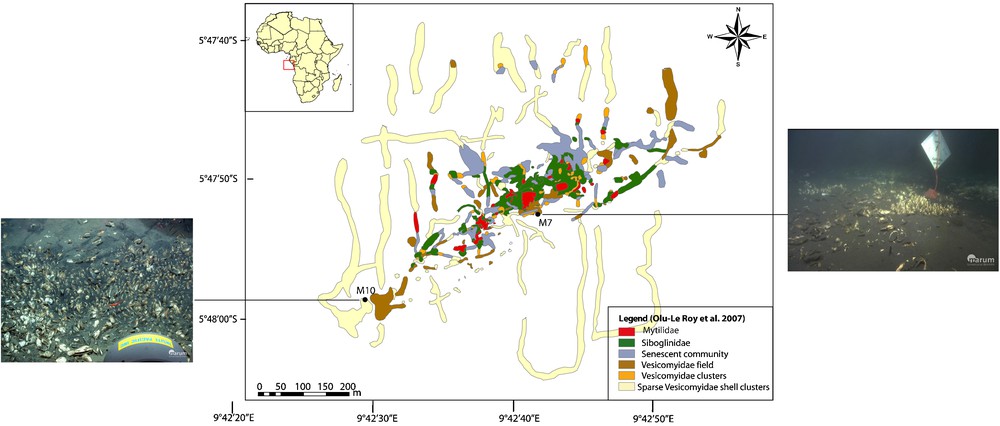
Location of the two study sites in the Regab pockmark: Site 1 at the centre of the pockmark (Marker GUINECO 7, M3 [26]) and Site 2 in the south-western part of the pockmark (Marker GUINECO 10, VC [26]). Images ROV QUEST, MARUM University of Bremen.
2.2 Benthic chamber deployment and data acquisitions
To assess in situ TOU and methane fluxes, the CALMAR benthic chamber [21] was deployed by the ROV on one clam bed at each site. CALMAR is a cylinder of 41 cm diameter opened at one end. It is equipped with six sampling cells controlled by a motor and a stirred to homogenize the water samples. The sampling sequence is programmed onboard, before the deployment. At each site, CALMAR was deployed during at least 6 hours (Fig. 2). Deployment time depended on the number of bivalves under the benthic chamber, previously estimated. In a 6-hour time lapse, oxygen concentrations in the CALMAR chamber could decrease and stabilise, making it possible to calculate oxygen fluxes. Penetration depth was estimated from video-monitoring of the ROV and the corresponding volume of the chamber is used for flux estimates. The maximum volume of water enclosed under the chamber is 40 L. When the benthic chamber was placed, the ROV removed the magnet starter to start the sampling sequence.
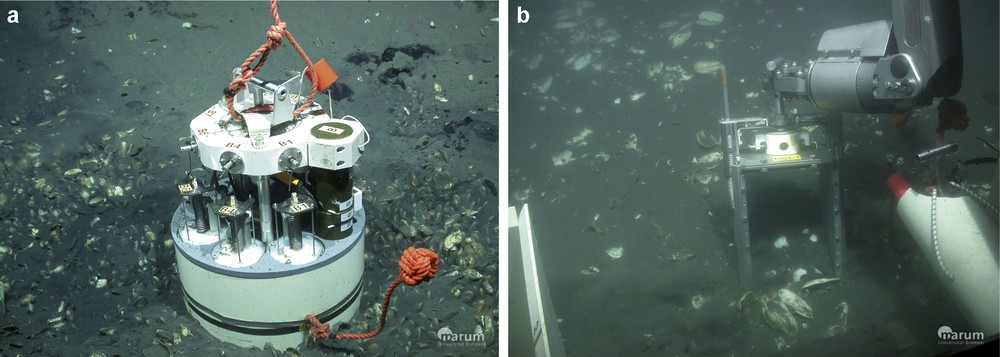
Deployment of the benthic chambers: a: the CALMAR benthic chamber positioned over a vesicomyid bed; b: sampling vesicomyids using a blade corer (images ROV QUEST, MARUM, University of Bremen).
Variations of oxygen and methane concentrations in the chamber were obtained by analysis of sequential water samples (6 sampling cells) and oxygen optode probe (Aadi®) measurements. The analytical methods are described in detail in Caprais et al. [21]. Briefly, dissolved oxygen concentration was analyzed in water samples using the modified Winkler titration method [27] with a precision of 2 μmol.L−1. Methane was analyzed by gas chromatography coupled with a headspace system (GC-HSS) [28]. Using the oxygen probe, in situ data can be obtained with better temporal resolution (data acquisition every 5 s) and a precision of 5%. The fluxes were calculated from the slope of the linear regression before the plateau, obtained with oxygen concentration versus time (Fig. 4). The plateau indicates a stop of oxygen consumption by the vesicomyid bivalves. The oxygen concentration of 35 μM (Site South-West) and 50 μM (site Centre) could be considered as the threshold of hypoxia [29]. Below these concentrations, vesicomyid bivalves could shift to anaerobic metabolism as demonstrate for costal bivalves or die [29].
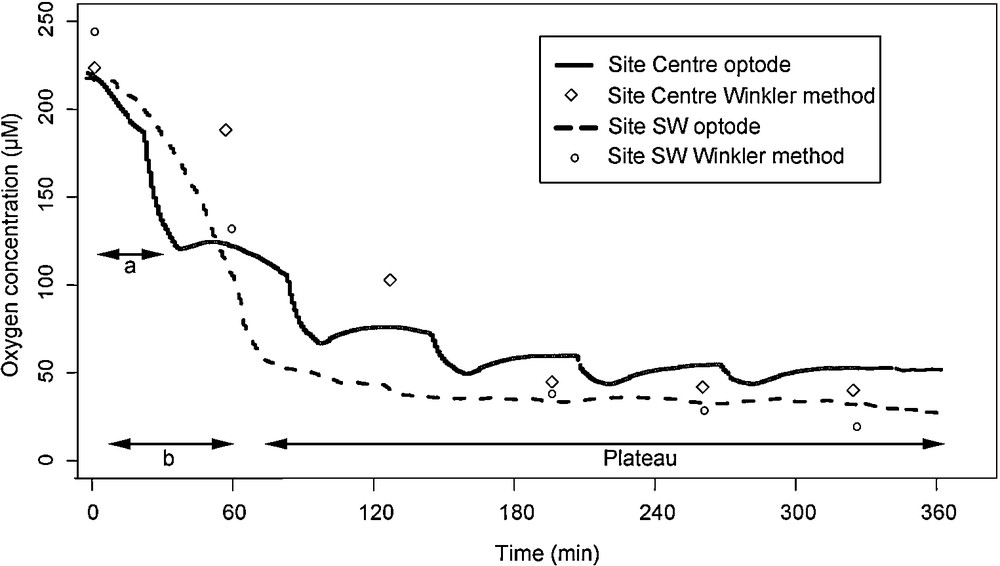
Time evolution of oxygen concentrations under the CALMAR benthic chamber on both beds, obtained using chemical (Winkler) analysis of sequential samples and an oxygen probe. a: linear regression used for flux calculation for Centre site; b: linear regression used for flux calculation for SW site.
Methane fluxes were also calculated from the slope of the linear regression obtained with methane concentration versus time (Fig. 5).
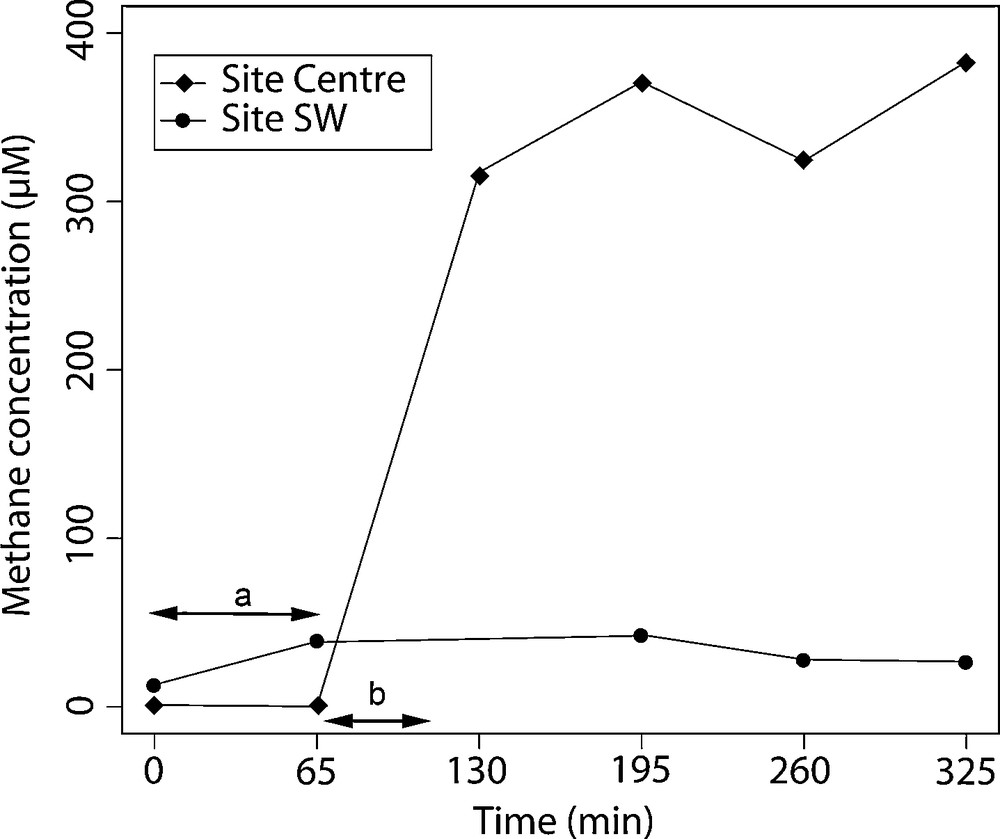
Time evolution of methane concentration under the CALMAR benthic chamber on both beds, obtained using the Winkler chemical method. a: linear regression used for flux calculation for SW site; b: linear regression used for flux calculation for Centre site.
2.3 Sampling and sample processing
Vesicomyid beds were visually selected during dives to ensure roughly equivalent bivalve densities. Bivalves were counted on images, based on their visible siphons, even though siphon length varies among species [25] (Fig. 3). Two or three blade cores (quantitative samples from 0.036 m2, 20 cm in length) were taken at each site (Fig. 2), one associated with the benthic chamber, and one or two others in beds with approximately the same density. We assessed the proportion of vesicomyid biomass relative to total macrofauna biomass by comparing densities. Including the additional net samples from nearby beds, a total of 231 vesicomyids were sampled (Table 1). After blade cores and nets were recovered, vesicomyid species were identified, washed with seawater and fixed in 4% buffered formalin. Two species were collected: C. regab – the dominant species with 223 sampled individuals – and L. chuni with only 8 individuals sampled in the SW site (Site 2). In the blade cores associated to CALMAR deployments, only 2 individuals of L. chuni were sampled at Site 2. Some bivalves (50% from Site 1 and 100% from Site 2) were transferred to 70% ethanol after 2 days for further study.
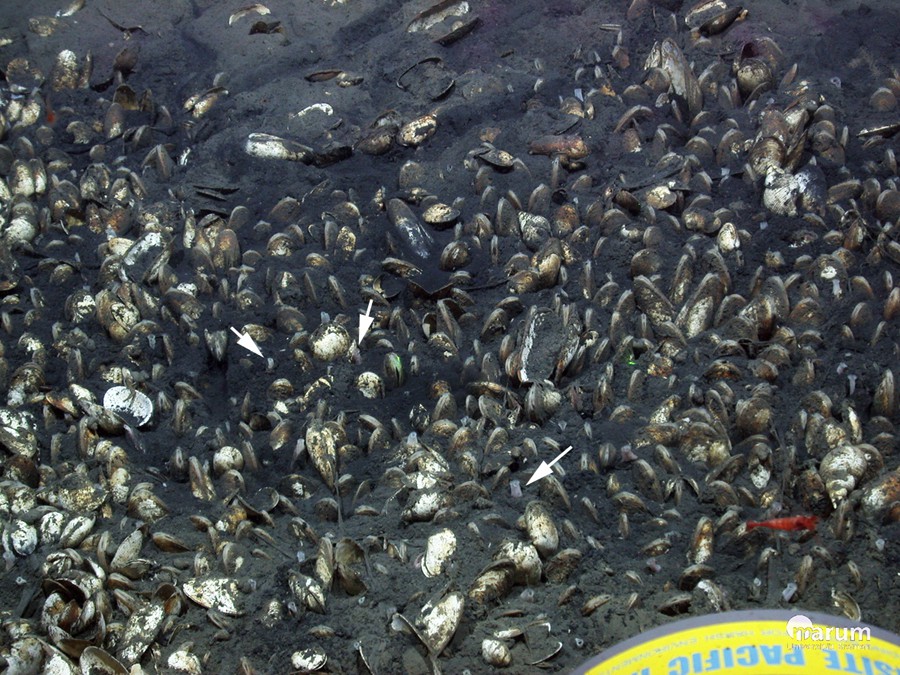
An aggregate of vesicomyid bivalve at site 2 (SW) with C. regab and L. chuni. Arrows show siphon of L. chuni (images ROV QUEST, MARUM, University of Bremen).
2.4 Density, biomass and condition index estimations
At each site, vesicomyid density (ind.m−2) was estimated using photos taken by the ROV before benthic chamber deployment and via quantitative sampling using blade cores (n = 2 or 3) (Table 2). Due to large standard deviations, stemming from differences obtained between these two methods (lower density based on image data compared to that estimated from blade cores) and from biases inherent to each method (abundance can be underestimated in images, surface sampled by blade core is smaller than that covered by the benthic chamber, and bed size can be smaller than chamber size), we chose to use both minimum and maximum densities to report a range of density and metabolism estimates.
Number of individuals weighed, minimum and maximum density and biomass estimations, and condition index at each site.
Site | Site 1-Centre | Site 2-South-West |
Number of individuals weighed | 39 | 29 |
Mean individual tissue wet mass (SD) (g) | 10.9 (3.3) | n.d. |
Mean individual tissue dry mass (SD) (g) | 2.2 (0.7) | 1.6 (0.4) |
Mean individual total dry mass (SD) (g) | 9.8 (2.3) | 8.6 (2.1) |
Mean individual AFDM (SD) (g) | 1.9 (0.6) | 1.5 (0.4) |
Condition index (SD) | 0.10 (0.3) | 0.08 (0.01) |
Mean density (SD) (ind.m−2) | 681 (296) | 1056 (218) |
Min (images) | 444 | 806 |
Max (blade cores) | 917 | 1277 |
Mean biomass (SD) (g tissue wet mass.m−2) | 7389.5 (3218.87) | n.d. |
Min | 3776.1 | n.d. |
Max | 9950.4 | n.d. |
Mean biomass (SD) (g tissue dry mass.m−2) | 1508.4 (657.1) | 1655.8 (341.5) |
Min | 770.8 | 1263.8 |
Max | 2031.2 | 2002.3 |
Mean biomass (SD) (g total dry mass.m−2) | 6699.0 (2918.1) | 9105.9 (1777.8) |
Min | 3423.3 | 6950.1 |
Max | 9020.5 | 11011.6 |
Mean biomass (SD) (g AFDM.m−2) | 1313.6 (572.2) | 1558.7 (321.4) |
Min | 671.3 | 1189.6 |
Max | 1768.9 | 1884.9 |
At the laboratory, total dry mass (shell + tissue), tissue dry mass, and ash-free dry mass (AFDM) were determined to the nearest 0.1 g for C. regab stored in 4% buffered formalin from Site 1 (39 individuals) and for a subsample of individuals stored in 70% ethanol from Site 2 (29 individuals). Dry mass was obtained after tissues and shells were dried for 24 h at 60 °C. Ash weight was measured after combustion for 12 h at 550 °C. AFDM was determined after subtracting ash weight from dry weight. Individual total mass and AFDM were then compared among sites using the Student's t-test. For each site, mean, standard deviation and minimum and maximum biomass (g total mass.m−2 and g AFDM.m−2) were calculated using minimum and maximum density, and mean individual total dry mass, tissue dry mass and AFDM. As L. chuni was not dominant [4 individuals counted under the benthic chamber (Table 1)], biomass estimates were based only on C. regab. Biomasses were compared among sites using non-parametric test (Wilcoxon-Mann-Whitney). Condition indexes (tissue dry weight/internal shell volume) [30] were estimated for individuals at each site (39 individuals from Site 1 and 29 individuals from Site 2). All analyses were performed using the free open-source R environment [31]. The Vegan library was used for the statistical tests [32].
2.5 Estimates of oxygen consumption rate
At each site, an attempt to estimate bivalve oxygen consumption rates (μmol.g total mass−1.h−1 and μmol.g AFDM−1.h−1) was made using TOU and biomass. The mean and standard deviation were also calculated. This value is considered as the upper estimate as experiment did not allowed one to assess the part of the TOU attributed to macrofauna and microorganism activities during the experiment with the CALMAR.
3 Results
3.1 Total oxygen uptake and methane fluxes
At both sites, oxygen measurements from both methods, sequential samples and oxygen probes, were correlated (R2 = 0.92) (Fig. 4). At Site 1, the oxygen concentration under CALMAR was 222.2 μmol.L−1 at the beginning of incubation. After 6 h incubation, the oxygen concentration was 51.0 μmol.L−1. Despite a stepwise decrease in concentration, most of the oxygen was consumed after about 2 h (Fig. 4). The “zigzag” observed was probably related to a lack of homogenization of water under the chamber. Indeed, the stopping of the stirrer motor during sequential water sampling could have been too long in this case, compared to the other deployment [21]. At Site 2, oxygen concentrations under the CALMAR benthic chamber decreased from 215.1 μmol.L−1 to 31.9 μmol.L−1 after 6 h incubation (Fig. 4). As in Site 1, Site 2's profile shows a sharp decrease in concentration after 2 h. TOUs, calculated from the slope of the optode profiles, were 332 mmol.m−2.d−1 at Site 1 and 492 mmol.m−2.d−1 at Site 2 (Table 3).
Total oxygen uptake, methane flux and respiratory rates estimates at each site.
Site | 1-Centre | 2-South-West |
Total oxygen uptake (mmol.m−2.d−1) | 332 | 492 |
Methane flux (mmol.m−2.d−1) | 14.6 | 0.3 |
Mean oxygen consumption rate (SD) (μmol.g tissue wet mass−1.h−1) | 2.2 (0.4) | n.d. |
Min | 1.4 | n.d. |
Max | 3.7 | n.d. |
Mean oxygen consumption rate (SD) (μmol.g tissue dry mass−1.h−1) | 10.9 (6.1) | 12.8 (2.8) |
Min | 6.8 | 10.2 |
Max | 17.9 | 16.2 |
Mean oxygen consumption rate (SD) (μmol.g total dry mass1.h−1) | 2.5 (1.4) | 2.3 (0.5) |
Min | 1.5 | 1.9 |
Max | 4.0 | 3.0 |
Mean oxygen consumption rate (SD) (μmol.g AFDM−1.h−1) | 12.5 (7.0) | 13.6 (2.9) |
Min | 7.8 | 10.8 |
Max | 20.6 | 17.2 |
At the beginning of incubation, the methane concentration was 1.1 μmol.L−1 at Site 1 and 13.1 μmol.L−1at Site 2. Six hours later, concentrations were much higher at Site 1 (383.0 μmol.L−1) than at Site 2 (26.8 μmol.L−1) (Fig. 5). In contrast to TOU, methane flux was higher at Site 1 than at Site 2 (respectively: 14.6 mmol.m2.d−1 and 0.3 mmol.m2.d−1) (Table 3).
3.2 Density, biomass and condition index
Densities estimated from images were lower than those estimated from blade cores. Combining both estimates provides upper and lower estimates of density for each site. From image and blade core data, vesicomyid density ranged from 444 to 1277 ind.m−2 with highest mean value observed at Site 2 (1056 ± 207 ind.m−2) and the lowest at Site 1 (681 ± 119 ind.m−2) (Table 2).
At Site 1, individual total mass (dry weight + shell) was significantly higher than at Site 2: respectively 9.8 ± 2.3 g and 8.6 ± 2.1 g (t-test: t = 1.97, P < 0.053). Similar results were observed for AFDM (t = 3.61, P < 0.001) (Table 2). However, due to higher density, and despite lower individual weights, total biomass was higher at Site 2 (9.1 ± 1.9 kg total mass.m−2 and 1.6 ± 0.3 kg AFDM.m−2) than at Site 1 (6.7 ± 2.9 kg total mass.m−2 and 1.3 ± 0.6 kg AFDM.m−2), but this difference was not significant (Wilcoxon-Mann-Whitney test, total dry mass: W = 3, P = 0.28; AFDM: W = 5, P = 0.72). Significantly higher condition indexes were observed for individuals at Site 1 compared to Site 2 (Wilcoxon-Mann-Whitney test: W = 658.5, P < 0.001).
3.3 Oxygen consumption rates
At the centre of the pockmark (Site 1), the oxygen consumption rate estimated using the upper and lower biomass estimates and the TOU ranged from 1.5 μmol.g total mass−1.h−1 to 4.0 μmol.g total mass−1.h−1 (Table 3). On the bed at the south-western part of the pockmark (Site 2), oxygen consumption rates were slightly lower: 1.9 μmol.g total mass−1.h−1 to 3.0 μmol.g total mass−1.h−1 (Table 3), but the difference between the two sites was not significant (Wilcoxon-Mann-Whitney test, W = 4, P = 1).
4 Discussion
4.1 Total oxygen uptake in deep-sea environment
Our values of TOU (from 332 to 492 mmol.m−2.d−1) are the highest ever reported for deep-sea species from chemosynthetic environments. To date, the highest TOUs from deep-sea species have been measured at the Håkon Mosby mud volcano (HMMV) in siboglinid tubeworm fields (161 mmol.m2.d−1) [22] and at the Hikurangi margin, in ampharetid polychaete ones (117.7 mmol.m−2.d−1) [23]. However, polychaete biomass is probably lower than vesicomyid biomass. For example, on HMMV, biomass of siboglinid tubeworms ranges from 350 to 435 g.m−2 [33], which is 10 times lower than the vesicomyid value on Regab (Table 2). Other TOU measurements performed with benthic chambers are much lower. For example, at the Hikurangi margin (New Zealand), benthic chamber deployment provided a TOU of 65.2 mmol.m2.d for siboglinid polychaetes [23]. At the Napoli mud volcano (Mediterranean Sea), the CALMAR deployed on Lamellibracchia sp. and small mytilid bivalves Idas sp. revealed a TOU of 35 mmol.m−2.d−1 [21]. Measurements performed on a vesicomyid bed at Hydrate Ridge [20] also show lower TOUs (from 2.0 to 5.1 mmol.m2.d).
4.2 Estimates of respiration rate of vesicomyid bivalve from TOU
Vesicomyid bivalves are the most pervasive of symbiont-bearing taxa in deep cold-seep ecosystems, and occur at most known cold-seep sites. Their density can reach up to 1000 ind.m−2 and their biomass ranges from 10 to 30 kg wet weight m−2 [34–36]. They occur in a wide range of sulphide and methane concentrations: from 0 to 25 mM [37] and from 0 to 300 μM in pore waters [38]. While mytilids and siboglinids are limited to the central, most active area of the Regab pockmark, vesicomyids are observed in soft sediments in areas affected by methane seeps, covering about 2% of the central part where methane and carbonate concretions are abundant, and 14% of the periphery area, with lower methane concentrations (0.5 to 4.4 μM at the sediment-water interface) and only on soft bottoms [26]. Via their symbiotic bacteria, vesicomyids contribute to sulphide oxidation and oxygen consumption, also needed for their own respiration. The intensity of these gas exchanges depends on the density and biomass of organisms and their metabolism. Although vesicomyids dominated the biomass in clusters, small endofauna and microbes were associated with bivalve assemblages [39–41]. According to differences in individual sizes, and therefore in biomass, associated fauna likely contribute relatively little to oxygen uptake. In Regab vesicomyid clusters, associated macrofauna densities were estimated but not biomass, therefore we used data from another cold-seep site [37] to assess the contribution of macrofaunal biomass: at the Cascadia margin vesicomyids accounted for more than 90% of the total macrofaunal biomass in vesicomyid beds. In the Regab pockmark, Site 1 showed higher macrofaunal densities (excluding vesicomyids) of 15,583 ind.m−2 (unpublished data) than those observed at the Cascadia margin (4968 ind.m−2), but vesicomyid densities were higher at Regab (this study, Table 2). Taking into account the macrofaunal biomass/density relation of Sahling et al. [37], macrofauna at Site 1 accounted for less than 5% of total biomass. At Site 2, macrofaunal density was similar to that observed on the Cascadia margin but vesicomyid density was higher: respectively, 3370 ind.m−2 (unpublished data) and 1056 ind.m−2 (Table 2). The bivalve therefore accounted for more than 95% of total biomass. The TOUs measured using the CALMAR chamber on vesicomyid beds (332–492 mmol.m−2.d−1) were more than 20 times higher than typical sediment just outside Regab (3.6 mmol.m−2.d−1 at 250 m from the Regab pockmark [42]) and in the eastern Mediterranean mud volcano cold-seep sediment beside siboglinids (13.5 mmol.m−2.d−1) [21]. These TOU measured are likely mainly due to endofauna which densities were less than 2000 ind.m−2 outside Regab [39] and 2900 ind.m−2 in the mud volcano sediments [43]. We therefore assumed that the TOU used for endofauna respiratory was negligible compared to the bulk used for vesicomyid oxygen consumption.
Oxygen is also consumed by microbes in the sediment [19,20,44,45]. Nevertheless, Sommer et al. [20] have measured that on vesicomyid beds of Hydrate Ridge, aerobic oxidation of methane was negligible. Moreover, they have found that more than 83% of TOU were used for sulphide oxidation and the rest for ammonia and organic carbon mineralization. As vesicomyid clams contain sulphide-oxidizing symbionts, we can assume that 83% of TOU was used for their metabolism. Then, oxygen can also be consumed by the chemical oxidation of sulphide. Nevertheless, as indicated by Luther et al. [46], the chemical oxidation of sulphide rate is at least three orders of magnitude lower than the microbial anaerobic or aerobic oxidation rate.
Taking into account that 17% of TOU was used for microbial processes at both sites and 5% for endofauna respiration, TOU induced to vesicomyid oxygen consumption is 259 and 384 mmol.m−2.d−1 respectively at Sites 1 and 2. A lower estimation of vesicomyid respiratory rate therefore could be 1.9 ± 0.4 and 1.8 ± 0.4 μmol.g total dry mass−1.h−1 respectively and 1.7 ± 0.3 μmol.g wet tissue mass−1.h−1 for Site 1 (Table 4).
Total oxygen uptake from sulphide oxidation (83.4% of TOU) without endofauna respiration (5% of TOU) and lower estimates of respiration rate from these values of TOU from sulphide oxidation.
Site | 1-Centre | 2-South-West |
Total oxygen uptake from sulphide oxidation (mmol.m−2.d−1) | 259 | 384 |
Mean oxygen consumption rate (SD) (μmol.g tissue wet mass−1.h−1) | 1.7 (0.3) | n.d. |
Min | 1.1 | n.d. |
Max | 2.8 | n.d. |
Mean oxygen consumption rate (SD) (μmol.g tissue dry mass−1.h−1) | 8.5 (1.7) | 10.0 (2.1) |
Min | 5.3 | 7.9 |
Max | 14.0 | 12.6 |
Mean oxygen consumption rate (SD) (μmol.g total dry mass−1.h−1) | 1.9 (0.4) | 1.8 (0.4) |
Min | 1.2 | 1.4 |
Max | 3.1 | 2.3 |
Mean oxygen consumption rate (SD) (μmol.g AFDM−1.h−1) | 9.8 (1.9) | 10.6 (2.2) |
Min | 6.1 | 8.5 |
Max | 16.1 | 13.4 |
4.3 Comparison of respiration rate estimates of vesicomyid bivalves with deep and shallow species
The ex situ experimentally obtained oxygen consumption rate of the vent species C. magnifica Boss and Turner, 1980 [12] from the Galapagos Rift ranges from 0.4 μmol g tissue wet weight−1 h−1 at 2.1 °C to 1.58 μmol.g tissue wet weight−1 h−1 at 8 °C [8]. The consumption of the other vent species C. pacifica Dall, 1891 and C. elongata Dall, 1916 is lower (average 0.2 μmol g tissue wet weight−1 h−1 [10,11]. In comparison, our lower estimates were about 1.7 μmol g tissue wet weight−1 h−1 at about 2.6 °C. These oxygen consumption rates were higher than those of other species measured at similar temperature (e.g. C. pacifica) and close to the upper estimate obtained for C. magnifica at higher temperature.
Ex situ respiratory rate of the deep-sea bivalve Acesta excavaca is lower than for vesicomyid bivalves living in the Regab pockmark (respectively 5 μmol.g tissue dry weight−1 h−1 and 8.5 to 10.0 μmol g tissue dry weight−1 h−1 at 9 °C) [47] (Table 4). For comparison with shallow-water bivalves, ex situ measurements of oxygen consumption at 20 °C for Tapes philippinarum and Crassostrea gigas are higher than for deep-sea species, with values from 30 to 40 μmol g tissue dry weight−1 h−1 and 11 μmol.g tissue wet weight −1 h−1, respectively [48,49]. Finally, Arp et al. [8] observed similar oxygen consumption rates between C. magnifica and shallow-water bivalves.
4.4 Variation of TOU and respiration rate estimates of vesicomyid bivalve along the Regab pockmark
Although both CALMAR measurements were performed within the same pockmark (distance 430 m) and in the same biogenic habitat, TOU was different: 332 vs. 492 mmol.m−2.d−1. Differences in TOU may likely be not attributed to microbial process as microbial community and especially the number of AOM aggregates producing sulphide do not vary between sites populated by vesicomyids within Regab [40]. Small macrofauna density was higher at Site 1 than at Site 2 (unpublished data) and therefore cannot explain higher TOU at Site 2. These results can be therefore related to density differences of the two vesicomyid beds: 681 ± 297 ind.m−2 and 1056 ± 206 ind.m−2 at Sites 1 and 2, respectively. However, taking into account mean individual vesicomyid biomass, and given that individuals from Site 1 were larger than those from Site 2, and with higher dry weight for a given size, the average oxygen consumption rate was similar in both beds. These results show the importance of estimating biomass using indicators other than density to compare TOUs. Moreover, these estimations of TOU suggest that the oxygen consumption rates are relatively constant for a species within a given cold-seep. Condition indexes appeared to be related to metabolism, with individuals in good condition (Site 1) having the highest oxygen uptake.
4.5 Influence of habitat geochemistry on the biology of vesicomyid bivalves in Regab pockmark
Methane fluxes in vesicomyid clusters varied along the pockmark with higher values at the centre (14.6 mmol.m−2.d−1) than at the periphery (0.3 mmol.m−2.d−1), as observed with concentrations at the REGAB pockmark in 2001 (3.9 μM vs 0.65 μM) [26]. Other measurements on vesicomyid clam beds [20] show methane fluxes of 0.2–1.1 mmol.m−2.d−1, which are similar to the lowest fluxes at Regab. Heterogeneous methane fluxes have also been observed at Napoli [21] and HMMV [22], Costa Rica convergent margin [19] and among habitats at the Hikurangi margin [23]. In deep-sea sediment, AOM consortia oxidize methane and produce sulphide [9]. These consortia were found in sediment below vesicomyid beds [40]. With the assumption that, within the same habitat type (here vesicomyids) methane is a proxy of sulphide, methane flux heterogeneity may reflect sulphide flux heterogeneity.
At the Regab pockmark, methane flux heterogeneity is also observed within the same biogenic habitat (vesicomyid beds) and could also explain the difference in individual biomass and condition index for C. regab. For example, at Site 1 located in the centre, average individual total biomass and condition indexes were higher than at the peripheral site, Site 2 (9.8 ± 2.3 g and 8.6 ± 2.1 g, respectively).
To summarise, the site at the centre (Site 1) of the pockmark is populated by individuals of higher weight and in better physiological condition. Higher methane fluxes at this site support lower density of vesicomyids, but almost the same amount of biomass (tissue dry weight) than at the less active SW site; oxygen demands are thus similar between both sites.
Moreover, methane may also influence the vesicomyid species’ distributions, as previously observed [26]. At the centre, where the methane fluxes were higher, only C. regab was found, whereas in the south-western part of the pockmark, L. chuni was sampled, in addition to C. regab. This distribution can be attributed to differences in physiological adaptation, e.g. sulphide-binding ability and symbiont energy turnover as demonstrated for C. pacifica and C. kilmeri inhabiting cold-seeps in Monterey Bay [50,51]. Differences in soft part morphology between the two vesicomyid species at Regab, with longer siphons observed for L. chuni may also influence their distribution [25]. This second species was unfortunately too rarely observed to estimate its respiration rate, but it could differ from the dominant C. regab.
Disclosure of interest
The authors declare that they have no conflicts of interest concerning this article.
Acknowledgements
We are grateful to the chief scientist of the GUINECO (MARUM) cruise (A. Boetius, MARUM); to the captain and crew of the R/V Meteor expedition M76/3b and the crew of the ROV Quest 4000 (MARUM). The GUINECO cruise was done with the support of the DFG Research Center The Ocean in the Earth System – MARUM – Center for Marine Environmental Sciences at the University Bremen. We are grateful to Frank Wenzhöfer for support with work at sea and to Christian Le Gall for the oxygen and methane measurements. English usage was professionally edited by Carolyn Engel-Gautier. We thank the two anonymous reviewers for their comments that help us to improve the manuscript.