1 Introduction
Estrogens are sex hormones involved in the development and maintenance of normal reproductive functions [1–3]. These hormones exert their biological effects through the interaction of two primary estrogen receptors, members of the nuclear steroid receptors superfamily: estrogen receptor-alpha (ERα), and estrogen receptor-beta (ERβ).
17β-Estradiol (E2) is the most potent and predominant form of estrogen; besides its recognized role in reproduction, it shows a number of effects on cognition and brain function [4–7]. In different brain regions, aromatase activity catalyzes E2 synthesis from androgen precursors and/or cholesterol. E2 is neurotrophic and neuroprotective and some recent results, although controversial, suggest that E2 synthesized within hippocampus and prefrontal cortex may contribute to the normal processes of memory consolidation [8–11]. The effects of E2 on several brain processes decline with age, including the ability to preserve the blood–brain barrier and to protect from stroke and neuroinflammation [12,13].
Accordingly with E2 functions, both ERα and ERβ subtypes are widely distributed in the brain regions, in particular in the hippocampus, the frontal cortex, and the amygdala [4,14]. The alteration in the normal distribution of ERs has been observed in hippocampal neurons of Alzheimer's disease (AD) patients [15].
It has been demonstrated that the two ER subtypes play different roles in E2-regulated gene expression and neuronal physiology [16]; the differential activation of ERα or ERβ is able to influence the expression of neuroprotective genes [17,18].
Data on the differential expression of ER genes in the central nervous system (CNS), mostly achieved in females and collected in the rat, the mouse or the human, are often contradictory [19–21]. In the rat hippocampus, ERα is transcriptionally more active than ERβ [7], and a significant decrease in the number of neurons positive to estrogen receptors during aging is observed [22]. In addition, ERs seem to have a gender specificity; indeed, ERβ expression in the female hippocampus is greater than in the male one [23]. In the cerebral cortex of the same animals, the limited data available show ERβ as the predominant receptor subtype immunolocalized [24].
In mouse, ERα is the predominant subtype in the hippocampus and in most of hypothalamus, whereas in the cerebral cortex ERβ is the main receptor subtype observed [25]. Conversely, in human and primates ERβ is the primary receptor reported in hippocampus [26,27]. In humans, it has been found that following the neurological diseases influencing memory, such as AD, the expression of ERα decreases while ERβ increases [28–31].
The relationship between E2 and ERs in the brain involves the regulation of food intake. In women, the daily food intake changes in relation to the E2 secretion during the menstrual cycle: during the follicular phase, the daily food intake is lower in respect to the luteal phase [32,33]. Also in rat, the increase in plasma E2 concentration during the preovulatory phase is associated with a transient decrease in food intake [34]. In the CNS, both ERα and ERβ are expressed in areas associated with satiety and feeding, such as hypothalamus and pituitary [35]; it has been also demonstrated that ER activation is involved in the metabolic effect of insulin signaling in the brain [36]. Finally, E2 has been proposed to act directly and indirectly to decrease orexigenic or anorexigenic peptides and food intake: for instance, ERα null mice are obese, insulin resistant, and show decreased energy expenditure [35,37].
In this study, by using Real-Time PCR analysis, we investigated the expression pattern of ERα and ERβ genes during aging and after a high fat diet (HFD) in rat brain. In particular, we evaluated the ERα and ERβ expression profiles in the cerebral cortex and hippocampus of pubertal young (2-months), adult (4- and 8-month), and middle-aged (16-month) rats. Our results demonstrate that ERα and ERβ subtypes have specific tissue- and age-related expression patterns that are unbalanced by an HFD that leads to the obese phenotype.
2 Materials and methods
2.1 Animal and experimental design
Male Wistar rats (Charles River, Calco, Como, Italy) of 2 months of age (adolescent), with the same starting body weight (160 ± 10 g), were individually caged in a temperature-controlled room (23 ± 1 °C) with a 12 h light/12-h dark cycle. Animals were housed in the Animal Care Facility at the Department of Biology, with ad libitum access to water and to a standard diet (SD, 10.6% fat J/J, 60,4% carbohydrate, 15.47 KJ/g) (Mucedola 4RF21; Settimo Milanese, Milan, Italy) up to 4 (social maturity, group 1), 8 (adulthood, group 2), or 16 (middle-aged, group 3) months. Six animals for each group were anesthetized with chloral hydrate (40 mg/100 g body wt) and killed by decapitation. Group 4 was made of eight adolescent rats sacrificed four days after their arrival in the animal facility.
In parallel, another group of rats (referred to as HFD, n = 12) received a high-fat diet rich in lard (40% fat J/J, 31% carbohydrate, 19.23 KJ/g). The HFD was formulated to differ from the standard diet in the fat and carbohydrate contribution to the energy value but to be identical in terms of proteins, vitamins, minerals and fibers [38]. Six animals of both SD and HFD groups were sacrificed after 4 weeks and other six individuals after 12 weeks. Throughout the experimental period, body weights and food intakes were monitored daily to calculate the body-weight gain. Spilled food was collected and compensated in readjusting the calculation of food intake.
At the end of the experimental period, animals of each group were anesthetized by chloral hydrate (40 mg/100 g body wt) and killed by decapitation. The brains were quickly removed and the cerebral cortex and hippocampus were dissected on ice. Samples of each brain region were snap frozen in liquid nitrogen immediately and stored at −80 °C for subsequent RNA isolation. The study was performed in strict accordance with the criteria established by the National Institutes of Health. The protocol for animal care and use was approved by the Committee on the Ethics of Animal Experiments of the University of Naples Federico II.
2.2 RNA purification and cDNA synthesis
Total RNA was extracted according to the TRI-Reagent (Sigma Aldrich) protocol. The quality of each total RNA was checked by electrophoresis on 2% agarose gel stained with ethidium bromide and measuring the optical density at 260/280 nm. A ratio of 1.8–2.0 was accepted for further reverse transcription. The QuantiTect Reverse Transcription Kit (Qiagen) was used for the removal of genomic DNA contamination and for the subsequent cDNA synthesis. Approximately 1 μg of total RNA was used, according to the kit's protocol.
2.3 Quantitative Real-Time PCR analysis
The Real-Time PCR reactions were carried out in triplicate for each sample in an Applied Biosystems 7500 Real-Time System by using the Power SYBR Green Master Mix PCR (Applied Biosystems) following the procedures recommended by the manufacturer [39]. Each SYBR Green reaction (20-μl total volume) contained 12 μL of Real-Time PCR Master Mix, 1 μL of each of the forward and reverse primer (10 μM), 2 μL of cDNA diluted 1:1 and 4 μL of nuclease free water. For internal standard control, the expression of the β-actin gene was quantified [40]. A single pair of specific primers for both ERα and ERβ isoforms was designed on the nucleotide sequences of Rattus norvegicus ERα (NM012689.1) and ERβ (AF042058.1); β-actin primers were designed on the R. norvegicus template (NM031144.2). Primer sequences are reported in Table 1. The lengths of the obtained cDNA fragments were: ERα, 216 bp; ERβ, 262 bp; β-actin, 128 bp. The PCR was performed under the following conditions: holding stage of 95 °C per 10 min; cycling stage (45 cycles): 95 °C × 10 s–60 °C × 10 s–72 °C × 10 s; melting stage: 95 °C × 5 s–65 °C × 1 min–95 °C × 30 s–40 °C × 30 s. The melting curve analysis of PCR products was performed in order to ensure gene-specific amplification. Changes in the gene expression relative to the different samples were calculated according to the standard 2−ΔΔCt method described by Livak and Schmittgen [41].
Specific primers used for Real-Time PCR analyses.
Name | Nucleotiode sequence (5’–3’) | Length (mer) |
ERα_F | GCACATTCCTTCCTTCCGTC | 20 |
ERα_R | CTCGTTCCCTTGGATCTGGT | 20 |
ERβ_F | ACAGTCCTGCTGTGATGAAC | 20 |
ERβ_R | ACTAGTAACAGGGCTGGCAC | 20 |
β-act_F | ACCCGCCACCAGTTCGCCAT | 20 |
β-act_R | CGGCCCACGATGGAGGGGAA | 20 |
2.4 Statistical analysis
Data are presented as mean ± standard error of the mean (SEM) from four separate experiments of each sample. Statistical analyses were carried out by GraphPad Prism software. The differences between the mean values were analyzed by one-way analysis of variance (ANOVA), followed by Fisher's LSD test. The differences were considered significant when p < 0.05.
3 Results
3.1 Expression of ERα and ERβ in cortical and hippocampal areas of 2 month-old rats
First, we investigated the relative abundance of ERα and ERβ in the cerebral cortex and hippocampus of young rats. We found that ERβ was more abundant in the cerebral cortex, whereas in the hippocampus ERα was dominant compared to the cortex (Fig. 1). In general, however, low but significant amounts of both ERα and ERβ were found in these brain regions.

Real-Time PCR analysis of ERα and ERβ expression in cerebral cortex and hippocampus of 2 month-old rats. ERα and ERβ mRNA levels are shown relative to the β-actin mRNA. The data represent the mean ± s.e.m.
Real-Time PCR analysis carried out in the cerebral cortex revealed a general decrease in ERα and ERβ expression during aging (Fig. 2). In particular, ERα transcripts decreased by half in 4 month-old rats, then the amount of transcripts remained constant; the ERβ transcript amount rapidly decreased to about one-tenth in 4 month-old rats, before rising in middle-aged rats at a fifth of the value measured in young rats (Fig. 2).

Effect of aging on ERα and ERβ gene expression in rat cerebral cortex (A) and hippocampus (B). The ERα and ERβ mRNA expression was normalized to that of β-actin mRNA and converted in fold change, compared with the 2 month-old rats. The data represent the mean ± s.e.m. Significance of differences is shown. *p < 0.05, **p < 0.001. a: significance vs. 2 months; aa: significance vs. 4 and 8 months; aaa: significance vs. 8 month.
In the hippocampus, the expression of ERα greatly increased with aging, whereas ERβ expression generally decreased (Fig. 2). Compared to 2 month-old rats, the level of ERα transcript was almost unchanged in 4-month-old rats and sharply increased by about 5 and 25 times in adult (8-month-old) and middle-aged (16 month-old) rats, respectively (Fig. 2). As regarding ERβ, a significant decrease in expression was observed in 4- and 8 month-old rats, with mRNA levels 1/5 lower than in 2 month rats; in middle-aged rats, the ERβ expression level slightly increased, although remaining below the value observed in young rats (Fig. 2).
3.2 HFD-related changes of ERα and ERβ expression in rat brain
As no data are available on the effect of a HFD on the brain expression of ERs, we evaluated ERα and ERβ mRNA levels in cortex and hippocampus of rats fed a HFD for 4 or 12 weeks.
First of all, we determined the weight gain of HFD-fed rats. Both control and HFD rats showed a significantly weight gain during housing (Table 2). The increase in body weight was affected by the duration of the housing and diet administration. In control animals, the weight gain was of 18% after 4-week and 35% after 12-week housing, respectively. In HFD rats, the weight gain was 47% after 4 weeks and about 80% after 12 weeks, with an increase by 25% between the two 4-week groups and of 48% in the 12-week group.
Average body weight in control and HFD rats.
Control | High fat diet | ||||
0 weeks | 4 weeks | 12 weeks | 4 weeks | 12 weeks | |
Body weight (g) | 311.47 ± 14.3 | 369.22 ± 15.0 | 422.69 ± 18.9 | 460.17 ± 32.1 | 615.4 ± 41.2 |
Weight gain (%) | 18.5 | 35.7 | 47.7 | 97.5 |
ER expression, as detected by Real-Time PCR, was significantly affected by the diet. In the cortex of HFD rats receiving the diet for 4 weeks, the level of ERα transcripts did not change, whereas the level of ERβ transcripts was lower than in control animals (about 1/5, Fig. 3). Interestingly, after 12 weeks of HF diet, both ERα and ERβ transcription was dramatically up-regulated (54-fold and 141-fold, respectively) (Fig. 3).
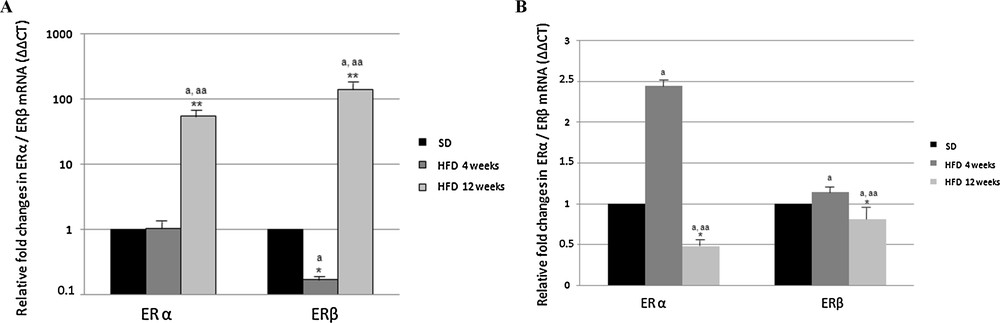
Effect of high fat diet on ERα and ERβ gene expression in rat cerebral cortex (A) and hippocampus (B). The ERα and ERβ mRNA expression was normalized to that of β-actin mRNA and converted in fold change, compared with rats fed with a standard diet. The data represent the mean ± s.e.m. Significance of differences is shown. *p < 0.05, **p < 0.001. a: significance vs. standard diet; aa: significance vs. high fat diet for 4 weeks.
In the hippocampus of rats receiving the HF diet for 4 weeks, the level of ERα transcripts increased about two-fold with respect to control rats, but decreased below the value determined in the control rats, when the treatment was extended for 12 weeks (Fig. 3). As regarding ERβ, the expression level was not affected by the HFD, no matter how long it was (Fig. 3).
4 Discussion
Over the past 25 years, evidence shows that estradiol modulates cognitive function in mammals, including humans. The E2 action begins in utero when estrogens direct sexual differentiation of the brain regions controlling reproduction and some cognitive functions linked to it. They reach the highest levels during puberty; with aging, levels of circulating estrogens collapse, contributing to the age-related declines in learning and memory function.
The aim of this study was to assess whether changes in ERs occur with aging in rattus cerebral cortex and hippocampus. Investigations were performed in males to avoid the influence of the fluctuations of steroids, mainly of E2, on the expression and localization of these receptors in brain [42–44].
First, our results demonstrate that the two ER subtypes are differentially expressed in the two brain regions examined. Indeed, regardless of the rats’ age, in cerebral cortex the ERβ subtype is more abundant with respect to the hippocampus; conversely, in the latter, ERα is more abundant. However, our results show also a very low transcription rate of both ERα and ERβ in rat brain. From these data, we can speculate that in male rats, ERβ is involved in the control of the complex mental functions of the cerebral cortex, whereas ERα may have a role in the hippocampus activities, such as learning and long-term memory. This hypothesis is corroborated by the findings showing that the learning performance in ERβ-KO mice was higher to that observed in ERα-KO, indicating that removal of ERβ preserves the learning abilities [44].
Many studies reported age-related changes in the transcript level of several genes in the mammalian nervous system [45,46]. We found that the relative abundance of ERα and ERβ transcripts changes during aging in both cortex and hippocampus. In the cortex, we observed a general and significant decrease in ER content from 2- to 16-month-old rats, and these findings are in agreement with that found in the cerebral cortex of mice, where ERα and ERβ transcription rate decreases in males with increasing age [42]. The ER expression trend in the hippocampus is different, as with aging ERα is up-regulated, while ERβ is down-regulated. In particular, in the male 4-month-old rats, the level of ERs does not change significantly compared to 2 month-old rats, but in adult (8-month) and middle-aged (16-month) rats, the ERα expression considerably increases by about 5 and 25 times, and the ERβ decreases by about 1/5 and 1/2, respectively.
On the basis of the current knowledge, the ER down-regulation observed in the cortical area could be linked to a neuroprotective effect [47]. It is known that Folstein test scores in women in AD terminal stage show an increase in ERα levels in the frontal cortex [48], and that estrogen and ER agonists are capable of altering the transcription of genes involved in neuroinflammatory responses in astroglia, interneurons and microglia of the frontal cortex [49–51].
Also in the hippocampus, the observed ERα up-regulation could have a neuroprotective role against the onset of neurodegenerative diseases, contributing to the effectiveness of the hormonal therapy ameliorating the memory loss that often accompanies advanced age [52]. In transgenic mice and in humans, it has been demonstrated that cytoplasmatic ERα inhibits the development of AD pathology [53,54], whereas ERβ increased in the hippocampal cells of AD patients [28]. Moreover, the expression of ERα seems to restore the protective action of estrogen against amyloid-β peptide, supporting the key role of ERα in estrogen-mediated neuroprotection [55].
Because ERβ may act as a negative regulator of ERα-mediated transcription [7,22], a general low level of ERβ transcripts during aging in brain is not surprising.
Another objective of this work was to evaluate the expression of ERα and ERβ in brain of rats fed with an HFD for 4 and 12 weeks, a condition that is able to induce systemic and peripheral inflammation, insulin resistance, hepatic steatosis, higher serum glucose and leptin levels [56], as well as inflammation and decrease in the haptoglobin level in the brain [38]. Once again, from our data emerges a different response in the two brain regions. In cortex, the 4-week HFD treatment affects only ERβ expression, which significantly decreased; conversely, the 12 weeks of HFD induced a huge increase (by about 100-fold) in both ERα and ERβ expression. In the hippocampus, the ERβ transcripts level did not change following HFD, whereas ERα transcript level showed a two-fold increase after 4 weeks of treatment and a halving after 12 weeks.
Evidence exists that circulating estrogen levels are able to induce the expression of leptin receptors, probably through estrogen responsive elements present in the gene promoter region [57]. A crosstalk between ER and leptin receptors has also been evidenced [58–60]. For this reason, the weight gain observed in postmenopausal women is often associated with the decrease in estrogen levels occurring in this period. It has also been demonstrated that estrogen is involved in the regulation of ghrelin expression, so the ER down-regulation may jointly cause a reduced response to leptin and an increased susceptibility to ghrelin.
Further, it is interesting to note that obesity increases the risk of age-related cognitive decline and is accompanied by peripheral inflammation [61–63]. In particular, dietary obesity impaired hippocampus-dependent memory and reduced long-term potentiation [63].
In this scenario, it is conceivable that the huge increase in ER expression observed in the cortex of rats fed for 12 weeks with a hypercaloric diet may represent a defense mechanism to counterbalancing the excessive energy intake, increasing sensitivity to leptin and reducing hunger. Conversely, the lack of ER responsiveness to HFD in hippocampus might be one of the motives for which the dietary composition negatively affects hippocampal functions, making this brain area, crucial for learning and memory, one of the most vulnerable sites in inflammation, in early AD and in other neurodegenerative diseases development.
Taken together, our data add a new slice to identify factors that significantly change in the brain following aging and HFD administration and unveil another possible molecular mechanism involved in the brain consequences of dietary fats.
Acknowledgments
The authors are grateful to Prof. Lillà Lionetti and Prof. Luisa Cigliano for providing the two different rat brain regions.