1 Introduction
Like trees, dendrimers usually exhibit aesthetically pleasant structures. However, as in the case of a tree, the interest in a specific dendrimer does not depend on its beauty, but on the ‘fruit’ (i.e. specific function) that is able to produce [1]. For dendrimers the capability of performing a specific function derives from the possibility of incorporating in predetermined sites of their structure selected functional groups, i.e. ‘pieces of information’ in the form of specific properties such as absorption spectra, luminescence, redox levels, etc.
From a topological viewpoint, we can distinguish dendrimers containing functional groups in the core, in the branches, in the surface, in the core and in the branches, in the core and in the surface, in the branches and in the surface, and in the core, branches and surface (Fig. 1). Functional units can also be non-covalently hosted in the cavities of a dendritic structure [2] (type h in Fig. 1) or associated on the dendrimer surface [3] (type i in Fig. 1). Because of their proximity, the various functional groups of a dendrimer may easily interact with one another and, in any case, the resulting properties are modulated by the dendrimer as a whole. Therefore, dendrimers incorporating functional units can be considered as supramolecular species.
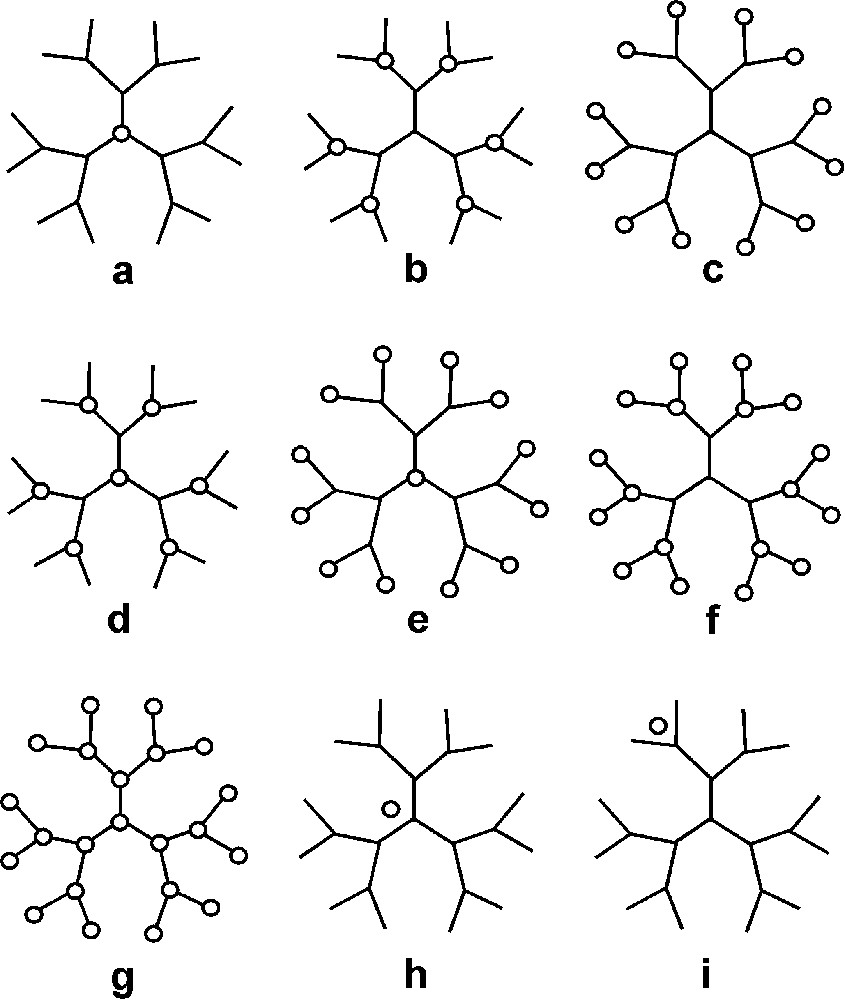
Schematic representation of dendrimers with positions where electroactive units (circles) can be located.
Dendrimers containing electroactive units as functional groups are very interesting species for several reasons. Potentially electroactive units can be buried in a dendrimer core, thus preventing the exchange of electrons. A very large number of equivalent, non-interacting electroactive units can be appended at the surface of a dendrimer, giving rise to the simultaneous exchange of a large and predetermined number of electrons. Electroactive units of the same or of different types can be located in topologically equivalent or not equivalent sites of a dendrimer to obtain made-to-order redox patterns. Furthermore, the interest in dendrimers containing electroactive units also relies on the fact that electrochemistry is a powerful technique (i) to elucidate the structure (and purity) of dendrimers, (ii) to evaluate the degree of electronic interaction of their chemically and/or topologically equivalent or not equivalent moieties, and (iii) to study their endo- and exo-receptor capabilities.
For space reasons, in this article we will only review the behaviour of dendrimers containing electroactive units of the type a and g of Fig. 1 with particular reference to the work carried out in our laboratory.
2 Electroactive units
An important requirement for any kind of application is the chemical redox reversibility of the electroactive units incorporated in dendrimers.
The most common metal complexes capable to exhibit a chemically reversible redox behaviour are ferrocene and its derivatives and the iron, ruthenium and osmium complexes of polypyridine ligands. Therefore, it is not surprising that most of the investigated dendrimers contain such metal-based moieties. In the electrochemical window accessible in the usual solvents, ferrocene-type complexes undergo only one redox process, whereas iron, ruthenium and osmium polypyridine complexes undergo a metal-based oxidation process and at least three ligand-based reduction processes. Other electroactive units that are often incorporated in dendrimers are free and metal-based porphyrins and phtalocyanins, iron-sulfur cluster, and organic molecules such as, for example, naphthalene diimide, tetrathiafulvalene, and fullerene. Recently, dendrimers bearing 4,4′-bipyridinium units, which can undergo two successive reversible one-electron reductions, have also been reported.
We will focus on dendrimers containing as electroactive units Ru(II) and Os(II) polypyridine complexes, ferrocene, and 4,4′-bipyridinium dication. For recent and exhaustive reviews on electroactive dendrimers see [4].
3 Dendrimers with an electroactive unit as a core
When the only electroactive unit of a dendrimer is that constituting the core of the structure (Fig. 1, type a), the most interesting problem is whether and, in case, how much the electrochemical properties (potential value, kinetic reversibility) of the core are modified by the surrounding branches.
For space reasons, we will only describe three families of dendrimers investigated in our laboratory and containing as a core a [Ru(bpy)3]2+-type, a ferrocene-type, and a 4,4′-bipyridinium-type units.
3.1 [Ru(bpy)3]2+-type core
[Ru(bpy)3]2+ (bpy = 2,2′-bipyridine) can be considered the prototype of the family of transition metal complexes of polypyridine-type ligands. This complex is one of the most investigated compounds in the last 40 years and its photophysical and electrochemical properties are well understood [5]. Fig. 2 shows its cyclic voltammogram in which seven redox processes are evidenced. The oxidation process corresponds to the oxidation of Ru(II) to Ru(III), whereas the two triplets of reduction processes correspond to the first and the second reduction of the three bpy ligands [6]. Since 2,2′-bipyridine can be easily functionalised in the 4 and 4′ positions, a variety of dendritic wedges can be appended to bpy ligands and dendrimers containing a [Ru(bpy)3]2+-type unit as a core can be prepared, such as dendrons 1 and 2 and dendrimers [Ru(bpy)2(1)]2+, [Ru(bpy)2(2)]2+ and [Ru(2)3]2+ shown in Fig. 3 [7]. The electrochemical experiments performed in acetonitrile solution on the small [Ru(bpy)2(1)]2+ dendrimer have evidenced six redox processes. By comparison with the behaviour of the model compound [Ru(bpy)2(Me2bpy)]2+, the three reduction processes (–1.35, –1.56, –1.81 V/SCE) and the oxidation process at less positive potential (+1.27 V/SCE), all reversible and monoelectronic, can be straightforwardly assigned to the metal complex moiety. The two oxidation processes observed at more positive potentials (+1.56, +1.80 V/SCE) can be assigned to the dioxybenzene- and oxybenzene-type units of the branches, respectively. Since these processes are not fully reversible, it is difficult to establish whether the number of exchanged electrons corresponds to that of all the electroactive units present in the wedge. As far as [Ru(bpy)2(2)]2+ is concerned, the first oxidation (metal-centred) and the first reduction (ligand-centred) processes occur at the same potentials as those of the smaller [Ru(bpy)2(1)]2+ compound. The potential values for the second and third reduction processes, however, cannot be determined because of adsorption phenomena on the electrode surface. Since such a phenomenon is not present in the fully dendritic [Ru(2)3]2+, it cannot be attributed to the peripheral units of the wedge and is likely related to the particular ‘comet shape’ of [Ru(bpy)2(2)]2+. On the oxidation side, the two not fully reversible multielectron processes involving the electroactive units of the wedge are also present. The area of the DPV peaks corresponding to these processes is larger than that observed in the case of [Ru(bpy)2(1)]2+, in agreement with the larger number of electroactive units present in the wedge of [Ru(bpy)2(2)]2+. For the fully dendritic [Ru(2)3]2+ compound, the two multielectron oxidation processes related to the numerous dioxybenzene- and oxybenzene-type units of the branches merge into a high and very broad DPV peak at +1.57 V/SCE. In CV experiments, such oxidation processes result in a huge wave that does not permit a detailed investigation of the core-based oxidation process. The potential value for this process (+1.26 V/SCE) can be obtained from DPV experiments and the corresponding peak seems to involve less that one electron. Comparison with the behaviour of the [Ru(Me2bpy)3]2+ model compound shows that oxidation and reduction occur at more positive potentials, and the observed processes are not fully kinetically reversible (as indicated by both CV and DPV for reduction, and from DPV for oxidation). Indeed, heterogeneous electron transfer rates usually decrease upon increasing encapsulation of the electroactive unit.

Electrochemical behaviour of [Ru(bpy)3]2+. The solvent is acetonitrile for the oxidation and dimethylformamide for the reduction processes.

Formulae of dendrons 1 and 2 and their corresponding dendritic metal complexes.
3.2 Ferrocene-type core
Ferrocene is the most commonly used electroactive unit for functionalisation of dendrimers [4g]. Its electrochemical behaviour is relatively simple, giving rise to a reversible monoelectronic oxidation process at a very accessible potential. Such a unit has been extensively used for functionalising the periphery of dendrimers [8], but examples of dendrimers containing ferrocene both as a core and as terminal unit [9] and as a core [10] are also reported.
Very recently, we have investigated [11] the electrochemical behaviour of two families of dendrimers (Fig. 4) containing a ferrocene core surrounded by protected (compounds 3, 5, 7 and 9) and deprotected (compounds 4, 6, 8 and 10) carbohydrate-based branches [10b]. In the potential window examined (–2/+2 V/SCE in MeCN for the protected compounds, –1/+1 V/SCE in H2O for the deprotected ones), all the dendrimers show the characteristic oxidation process of the ferrocene moiety and no reduction process. The diffusion coefficients, the potential for oxidation of the ferrocene core and the rate constants of the heterogeneous electron transfer have shown that the number (one or two) of substituents and the number (one or three) of carbohydrate branches present in the substituents play a crucial role in protecting the ferrocene core from solvent interactions, as well as from interactions with the electrode. Therefore, the examined species mimic the behaviour of proteins incorporating a redox active unit embedded into a polypeptidic framework. These electrochemical studies have also evidenced that the deprotected triply-branched dendrimer 10 exhibits a very peculiar behaviour. Its differential pulse voltammogram reveals two oxidation peaks the first one at +0.54 V and the second one at +0.64 V/SCE with an intensity ratio of approximately 1:2 (Fig. 5). This behaviour suggests that, in aqueous solution, derivative 10 is present in two distinguishable forms that experience different shielding effects at this electroactive ferrocene core. The reversible character of the two oxidation processes shows that the interconversion between these two species is slow on the timescale of the electrochemical experiments. The simplest hypothesis that accounts for the observed results is the existence of two conformers of 10 (Fig. 5), one having the two substituents placed on the same (cisoid) side of the ferrocene core and the other on opposite (transoid) sides. The cisoid form, which can be stabilized by intramolecular hydrogen bonds, will be less shielded from solvent interactions than the transoid form and will therefore be easier to oxidize. If this hypothesis is correct, the processes observed at +0.54 and +0.64 V/SCE correspond to the oxidation of the cisoid and transoid conformers of 10, respectively.
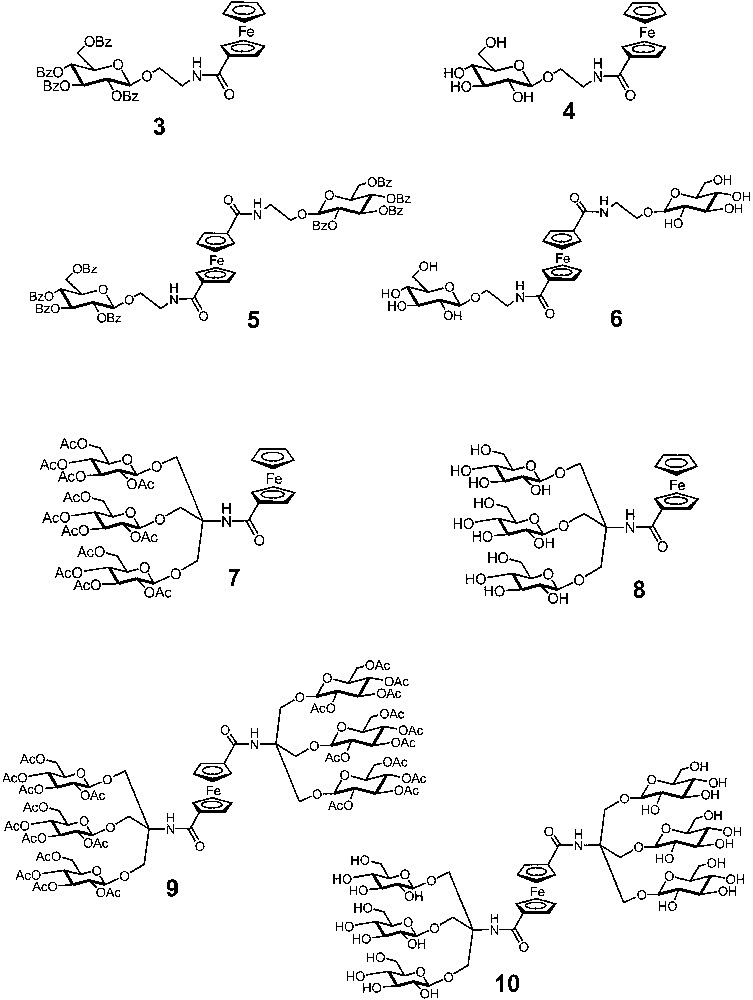
Formulae of ferrocene cored dendrimers surrounded by protected (3, 5, 7 and 9) and deprotected (4, 6, 8 and 10) carbohydrate-based branches.
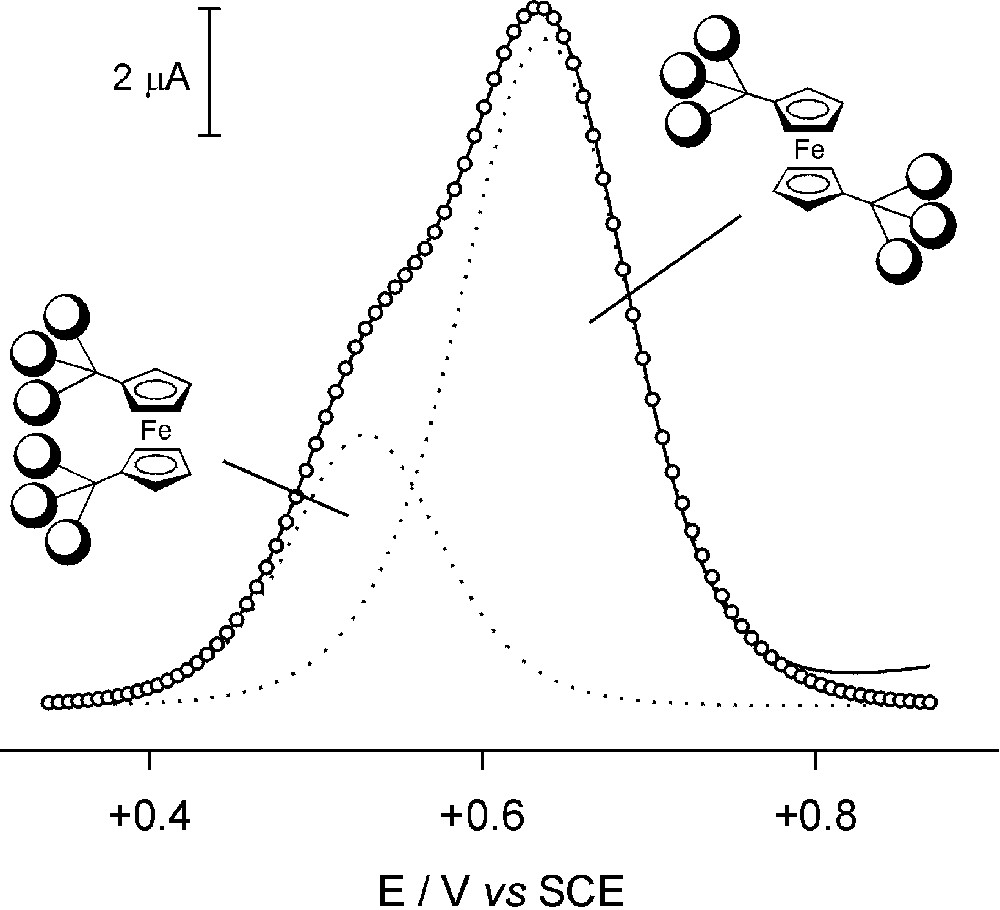
Differential pulse voltammogram of compound 10 of Fig. 4 (1 × 10–3 M, H2O, 0.1 M NaClO4, 22 °C; glassy carbon electrode, scan rate 20 mV s–1, pulse height 75 mV). The circles show a simulated DPV profile consisting of the sum of two individual peaks of different intensities (dotted lines).
3.3 4,4′-Bipyridinium-type core
4,4′-Bipyridinium-type units (usually known as viologens) are well-known electron acceptors [12] extensively used in chemical and electrochemical redox processes [13], since they can undergo two reversible one-electron reduction processes.
We have investigated the electrochemical behaviour of a family of dendrimers 11–13 (Fig. 6), constituted by a 4,4′-bipyridinium core surrounded by Fréchet-type dendrons from the first up to the third generation in acetonitrile solution [14,15]. The cathodic region shows two one-electron transfer processes reversible in all cases, except for 13. For the third generation dendrimer, the first cathodic peak is chemically and electrochemically reversible, while the second cathodic peak and the two anodic counterparts (particularly, the first one) present distorted shapes, due to adsorption on the electrode surface. In all cases, the two reduction processes can be straightforwardly assigned to the two successive reductions of the 4,4′-bipyridinium core, on the basis of the comparison with the 1,1′-dimethyl-4,4′-bipyridinium and 1,1′-dibenzyl-4,4′-bipyridinium model compounds. A small positive shift of the cathodic peak potential of the first reduction process is observed in the case of 13 compared to 11 and 12. This result suggests a relative destabilization of the dicationic core with respect to its reduced form, presumably caused by folding of the two larger dendrons around the 4,4′-bipyridinium unit. The electron transfer to the electrode surface is fast for all the dendrimers (in the case of 13, the first cathodic peak shows a Nernstian behaviour at scan rates up to 10 V s–1), thus indicating no significant inhibition or site isolation effect on the dendrimer core by the dendrons. This result is quite interesting since the isolation of the redox core by growing dendritic branches usually causes significant decrease in the rate of heterogeneous electron transfer. The anodic region presents two chemically irreversible oxidation processes assigned to the two one-electron oxidations of each 1,3-dimethyleneoxybenzene unit, as shown by the dendron of first generation. We have determined the diffusion coefficients of all the examined compounds by chronoamperometric experiments both with the standard glassy carbon electrode and with a Pt (r = 5 μm) ultramicroelectrode as working electrode. The diffusion coefficients decrease upon increasing the dendrimer generation, according to the equation D ∝ M–a, where D is the diffusion coefficient, M is the molecular mass of the compound, and a = 0.7 for the investigated dendrimer family. This equation is commonly used for macromolecules; in the approximation of a rigid homogeneous sphere a is close to 0.3 [16]. The experimental value observed in this case indicates a quite strong deviation from the spherical shape in the case of these dendrimers.
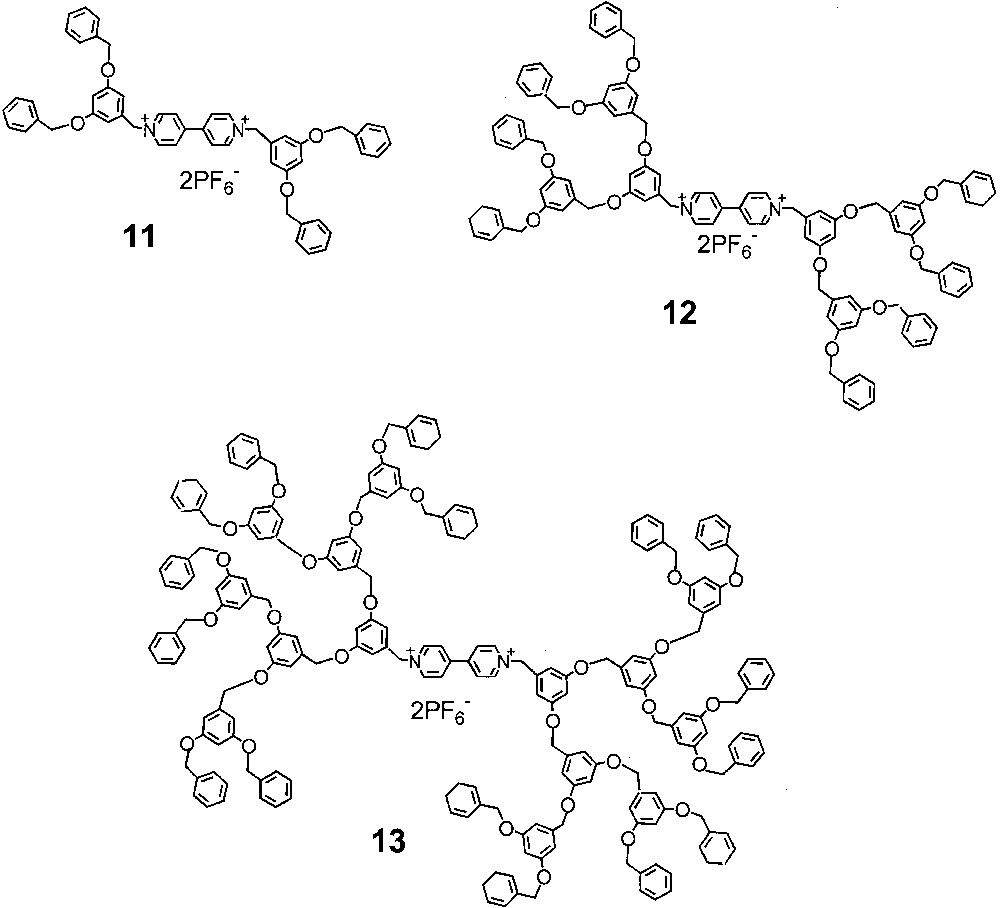
Formulae of 4,4′-bipyridinium cored dendrimers from the first up to the third generation.
4 Dendrimers with electroactive units in each branching centre
Most of the dendrimers containing electroactive units in each branching centre (Fig. 1, type g) are based on Ru(II) and Os(II) complexes of polypyridine-type ligands, but examples of dendrimers of this type containing up to 45 4,4′-bipyridinium units have also been reported recently [17].
We will only discuss the case of dendrimer based on metal complexes.
4.1 Ru(II) and Os(II) polypyridine-type complexes as branching centre
In this kind of dendrimers, the metal-containing units are linked together by bridging ligands. Fig. 7 shows the N-N bischelating 2,3- and 2,5-bis(2-pyridyl)pyrazine (2,3- and 2,5-dpp) bridging ligands and 2,2′-bipyridine or 2,2′-biquinoline (biq) terminal ligands that have been used to construct most of the dendrimers of this type [18].
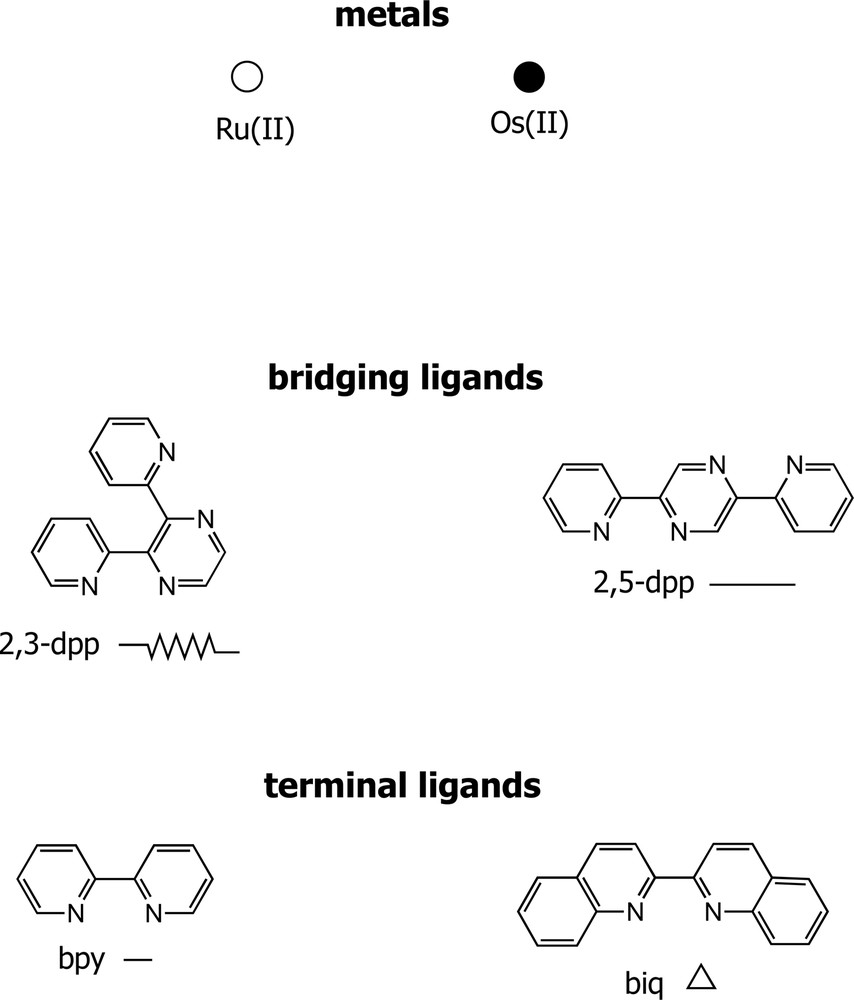
Formulae of ligands, abbreviations, and graphic symbols used to represent the components of the metal-based dendrimers of type g in Fig. 1.
Each dendrimer of this family can be viewed as an ordered ensemble of metal-based [M(L)n(BL)3–n]2+ units (M = Ru(II) or Os(II); L = bpy or biq; BL = 2,3- or 2,5-dpp) which are known [18] to exhibit reversible redox processes. For each unit, only one, metal-based, process is observed on oxidation in the potential window usually investigated (<+2 V/SCE). This process occurs at a potential which depends strongly on the nature of the metal ion [Os(II) is oxidized at less positive potentials compared to Ru(II)] and, less dramatically, on the nature of the coordinated ligands, whose electron-donor power increases in the series μ-2,5-dpp < μ-2,3-dpp < biq < bpy (alternatively, one can say that the electron-acceptor power decreases along the same series). The reduction processes are ligand localized and for each ligand the reduction potential value depends on its electronic properties and, to a smaller extent, on the nature of the metal and the other ligands coordinated to the metal. The first reduction potential becomes more negative in the ligand series μ−2,5-dpp < μ-2,3-dpp < biq < bpy. Each L ligand is reduced twice and each BL ligand is reduced four times, when coordinated to a metal ion, in the potential window –0.5/–3.1 V/SCE [19].
In the dendritic species each unit brings its own redox properties, more or less affected by intercomponent interactions. Metal–metal and ligand–ligand interactions are noticeable for metals coordinated to the same bridging ligand and for ligands coordinated to the same metal, whereas they are very small for metals or ligands that are sufficiently far apart. By placing in the dendrimer the desired number of suitable equivalents and noninteracting units, it is possible to control the number of electrons lost or gained at a certain potential.
The electrochemical features of this family of dendrimers are clearly illustrated by the oxidation patterns obtained for three strictly related decanuclear complexes (Fig. 8) in acetonitrile solution [20,21].
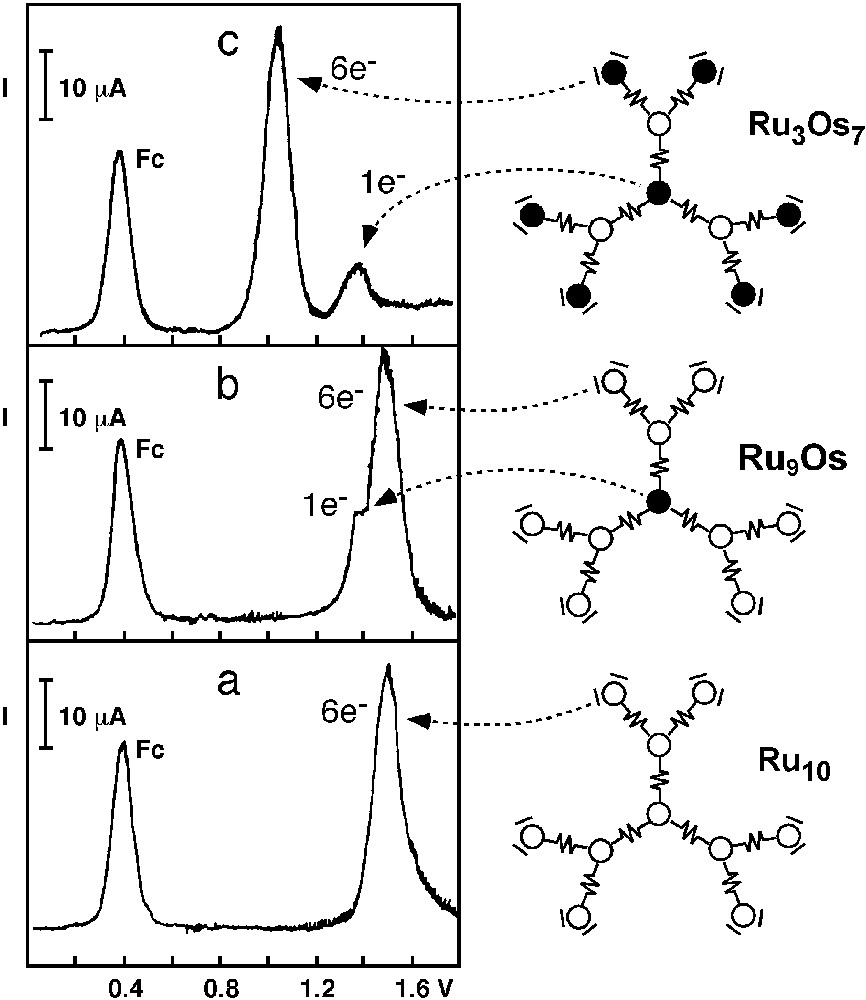
Oxidation patterns (differential pulse voltammetric peaks) for three decanuclear dendrimers. Peak Fc indicates oxidation of ferrocene used as internal standard.
In the [Ru{(μ-2,3-dpp)Ru[(μ-2,3-dpp)Ru(2,3-dpp)Ru(bpy)2]2}3]20+ compound (Ru10), the peripheral Ru-based units are expected to be oxidized at less positive potentials than the internal ones because the bpy ligands are better electron-donors than the bridging 2,3-dpp ligands. Furthermore, the six peripheral Ru-based units are not expected to interact with one another, because they are not directly connected. According with this expectation, the first oxidation process observed for this dendrimer (+1.53 V) involves the exchange of six electrons at the same potential (Fig. 8a). Oxidation of the intermediate and central Ru(II) ions is shifted toward more positive potential and cannot be observed in the accessible potential window.
In the [Os{(μ-2,3-dpp)Ru[(μ-2,3-dpp)Ru(2,3-dpp)2]2}3]20+ compound (Ru9Os), which is made of an Os(II)-based core and nine Ru(II)-based units, a 1–6 pattern is predicted for the electrons exchanged on oxidation. In agreement with these expectations, the differential pulse voltammogram of this compound (Fig. 8b) shows an oxidation peak at +1.35 V and a six-time higher peak at +1.55 V/SCE. On the basis of the potential values and number of electrons exchanged, the former peak can be assigned to the one-electron oxidation of the central Os(II) metal ion, and the latter to the simultaneous one-electron oxidation of the six peripheral noninteracting Ru(II) ions. Oxidation of the three intermediate Ru(II) ions is further shifted toward more positive potentials and cannot be observed in the accessible potential window.
For the [Os{(μ-2,3-dpp)Ru[(μ-2,3-dpp)Os(bpy)2]2}3]20+ compound (Ru3Os7), made of an Os(II)-based core, three Ru(II)-based units in the intermediate positions, and six Os(II)-based units in the peripheral positions, one expects that oxidation involves first the six peripheral Os(II) ions (which contain the stronger electron-donor bpy ligand in their coordination sphere), and then the central one, yielding for the electron exchange a 6–1 pattern instead than the 1–6 one observed in the previous case. This is fully consistent with the differential pulse voltammetry results which show an oxidation peak at +1.05 V six times higher than a following peak at +1.39 V/SCE (Fig. 8c). Following the same considerations made for the previous dendrimer, it is easy to understand why the oxidation of the intermediate Ru(II)-based units is not observed.
In the docosanuclear dendrimer made of an Os(II)-based core and 21 Ru(II)-based units, a one electron oxidation process at +1.42 V, assigned to the Os(II) ion, is followed by a 12-electron process at +1.54 V/SCE, due to the simultaneous oxidation of the 12 equivalent and non interacting peripheral Ru(II)-based units [21].
Oxidation of all metal centres can be observed only in a solvent that offers a wider potential window, like liquid sulphur dioxide at –70 °C [22]. For example, in the case of the above discussed decanuclear [Ru{(μ-2,3-dpp)Ru[(μ-2,3-dpp)Ru(bpy)2]2}3]20+ dendrimer, a 6–1–3 oxidation pattern is observed, which corresponds to the three electrochemically non equivalent sets of metal centres: (i) the six peripheral Ru ions (this oxidation process is observed also in acetonitrile, vide supra); (ii) the central metal unit; and (iii) the three intermediate and topologically equivalent Ru ions. The last set of metal centres is more difficult to oxidize compared to the core unit since they are directly linked to the peripheral oxidized Ru(III) ions.
Because of the presence of many polypyridine ligands, each capable of undergoing several reduction processes [19], the electrochemical reduction of these dendritic compounds produces very complex electron exchange patterns. For example, the previously seen [Ru{(μ-2,3-dpp)Ru[(μ-2,3-dpp)Ru(bpy)2]2}3]20+ compound shows a differential pulse voltammogram with two, broad peaks (at –0.73 V and –1.22 V/SCE) followed by several other overlapping peaks [23]. The first peak, which corresponds to the exchange of six electrons, is due to the one-electron reduction of the six outer equivalent bridging ligands. The width of the peak, compared to that observed on oxidation, suggests a non-negligible interaction between the two ligands coordinated to the same metal, resulting in two closely lying three-electron processes. The second broad peak, which involves three electrons, is assigned to the one-electron reductions of the three inner bridging ligands, occurring at close potential values. Since the interaction between reduced ligands depends also on the nature of the metal, the electron exchange pattern can be modified by replacing Ru(II) with Os(II) [21].
In conclusion, the electrochemical data offer a fingerprint of the chemical and topological structure of the dendrimers. Furthermore, the knowledge of the electrochemical properties of the [M(L)n(BL)3–n]2+ mononuclear component units and the synthetic control of the supramolecular structure allow us to design dendrimers with predetermined redox patterns. The made-to-order synthetic control of the number of electrons exchanged at a certain potential makes such polynuclear complexes very attractive in view of their possible application as multielectron-transfer catalysts [4a,24].
5 Conclusions
In the last 20 years, efficient methodologies for the synthesis of dendrimers have been developed. Nowadays, the main research activities are focused on the capability of dendrimers of performing specific functions which, in their turn, derive from the possibility of incorporating in predetermined sites of the structure selected functional groups (‘pieces of information’). In this regard, electroactive groups play an important role. Dendrimers with an electroactive core can be useful for modelling natural electroactive species, investigating the problem of insulating a unit from the electrode surface, and information processing. Dendrimers capable of exchanging large, well defined numbers of electrons according to a variety of predetermined patterns have already been used in the functionalisation of electrodes which can also work as sensors [4f,25], and will likely lead to applications in the fields of molecular batteries [4a,8b,26] and multielectron catalysis [4a,24]. Because of these and other not yet fully investigated properties, dendrimers containing electroactive units will become the object of increasing interest in the next few years.
Acknowledgements
Financial support from MIUR (Supramolecular Devices Project) and the University of Bologna (Funds for Selected Topics) is gratefully acknowledged.