1 Introduction
Proteins are amphiphilic and they use macromolecular scaffolds to control the presentation of functional groups at their surfaces. Given the versatility of proteins as functional materials in biological systems, it is desirable to understand and develop ways to control functional group presentation in synthetic amphiphilic macromolecules. Despite the fact that proteins are macromolecular in nature, most approaches toward mimicking proteins are small-molecule based [1]. Recently, polymeric scaffolds have been used for achieving biomimetic functions [2]. Since dendrimers are the only class of artificial macromolecules that can be obtained in high molecular weights in monodisperse form, the branched architecture is an excellent choice for biomimicry. The propensity of dendrimers to adopt a globular conformation at high generations reinforces this choice, since they could be pursued as possible globular protein mimics. Globular proteins are one of the most versatile architectures in nature, since they are involved in cellular chemical processes such as synthesis, transport, and metabolism [3]. The ability of these proteins to precisely perform complex cellular functions arises from the exquisite spatial presentation of appropriate functional groups in three-dimensional space.
Several reports exist on the use of dendrimer as an effective mimic of nature from an encapsulation standpoint [4]. In order to further enhance the versatility of dendrimers, our laboratory has initiated two research programs: (i) design of dendrimers in which the concave interior can be selectively functionalized; (ii) development of synthetic methodologies by which each monomer unit within a dendrimer can be varied, i.e., introducing functional group diversity. This account is organized in the following fashion. First, we will discuss the microenvironment of dendrimers using a few examples. Then, steric protection of functionalities through encapsulation will be discussed. The next section will give a very brief introduction to amphiphilic dendrimers and will discuss the monomer design criteria for achieving the dendrimers with a functionalized interior. The last section will deal with various synthetic approaches to diversify functional groups in dendrimers. Since the microenvironment of dendritic cores and encapsulation capabilities of dendrimers have been topics of recent reviews, the coverage of these two topics will not be exhaustive. However, discussion of these topics is warranted, since they serve as useful lead into our own research themes. Readers interested in aspects of encapsulation are referred to some of the recent reviews in this area [4].
2 Microenvironment of dendritic cores
Although dendrimers lack the functional group sophistication that proteins display in their surfaces, they do provide distinct microenvironments. Microenvironment of dendritic cores have been analyzed using techniques such as absorption and fluorescence spectroscopy [5], excited state lifetime measurements [6], and other techniques [7]. To analyze the microenvironment of dendrimers, Fréchet and co-workers synthesized benzyl ether dendrons with an N-methyl-p-nitroaniline moiety attached to the core [5a]. The solvatochromic property of this dye moiety reports the microenvironment of the dendritic core from the polarity perspective. This solvatochromic probe exhibited pronounced bathochromic shifts in nonpolar solvents at higher generations. In CCl4, the absorption maxima (λmax) shifted from 366 nm in a G–0 dendron to 383 nm in a G–6 dendron. The shift in the absorption maxima (Δλmax) was smaller in more polar solvents and was negligible in highly polar solvents such as DMF and DMSO. By plotting Δλmax against the solvent polarizability parameter (π*), the authors conclude that the higher generation benzyl ether dendrons provide a different microenvironment, the polarity of which is comparable to that of highly polar solvents such as DMF. An example of the dendrimer structure is shown in Fig. 1.
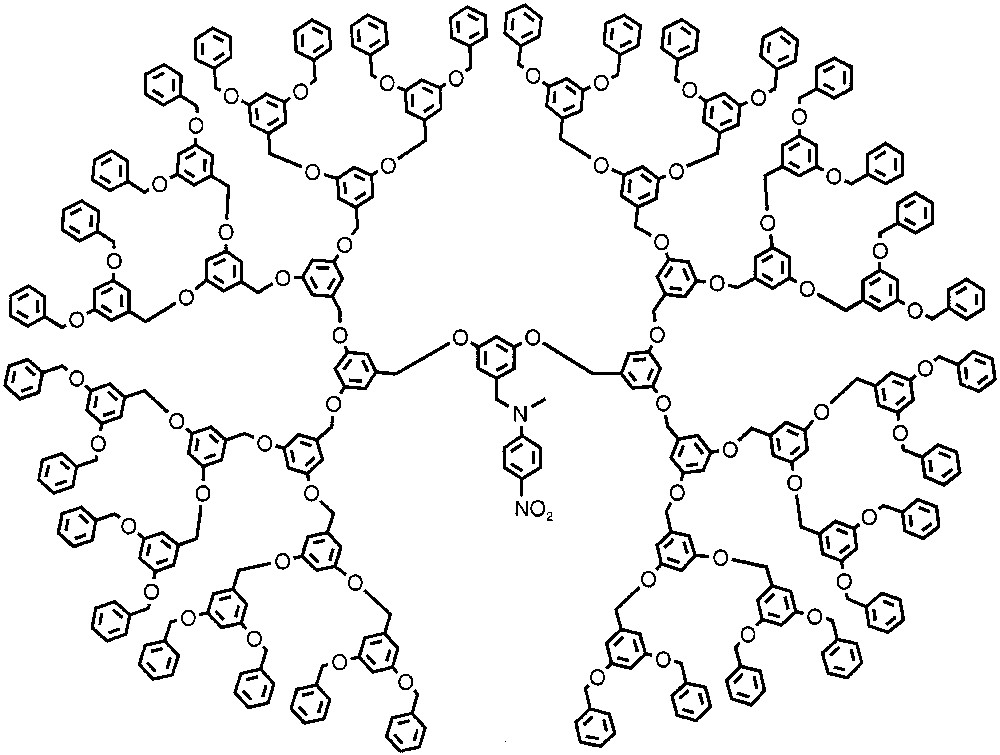
Benzyl ether dendron with p-nitroaniline moiety at the core.
More recently, Smith and Müller reported the emission behavior of a tryptophan chromophore with Newkome-type dendrimers (Fig. 2) [5c]. They noted that the dendritic effect is pronounced in non-hydrogen bonding solvents, whereas the effect is small to negligible in solvents that exhibit hydrogen bond acceptor or both hydrogen bond donors and acceptor characteristics.
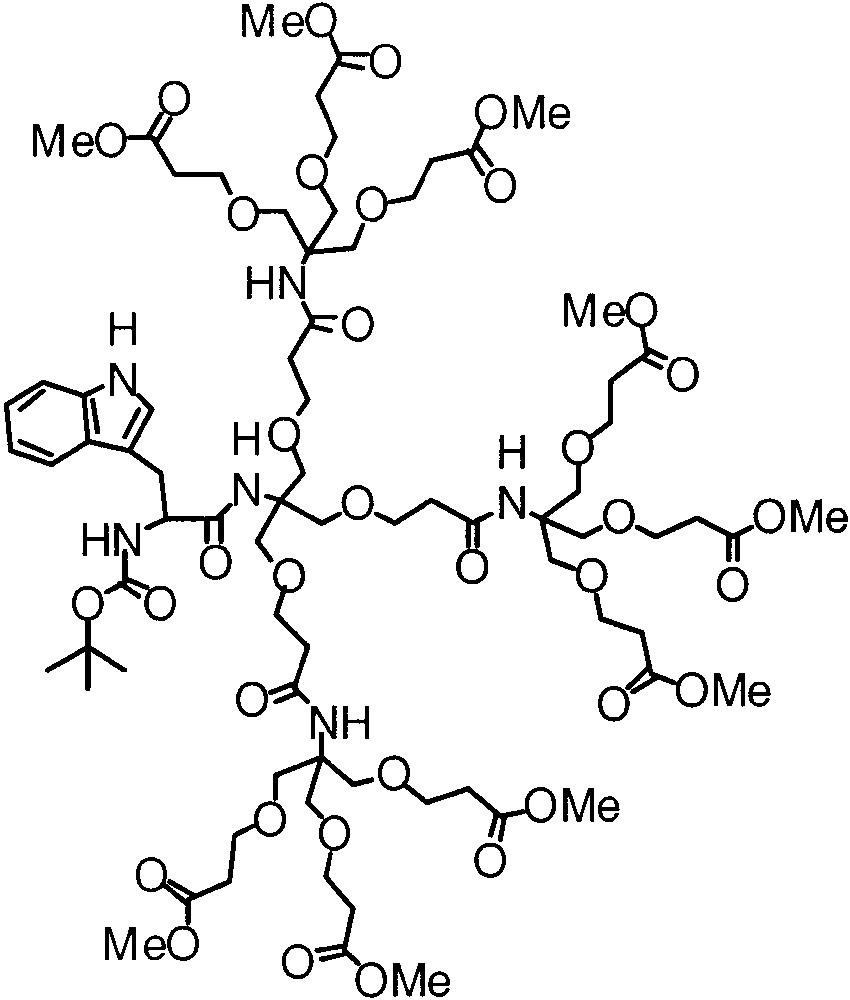
Dendritically encapsulated tryptophan.
Interestingly, Zimmerman and co-workers reported on the effect of dendritic substitution upon the hydrogen bonding based interaction between a naphthyridine host and a benzamidinium guest (Fig. 3) [7b]. The naphthyridine host moiety was incorporated as the core unit in benzyl ether dendrimers and phenylacetylene dendrimers. They noted that the binding constants were not affected significantly by the size of the didendrons. In fact, they report that the maximum δG0 for these host–guest complexes with different dendrimer generations was below 0.3 kcal mol–1. The complexation efficiency seems to depend heavily on the polarity of the solvent used and independent of the generation of the dendrimer. The authors, therefore, suggest that the dendrimers are highly porous in nature and have solvent-filled interiors. Thus, they also suggest that the microenvironment of the dendrimers is not different from the bulk solvent at least from the hydrogen bonding perspective. The results of Fréchet, Smith and Zimmerman groups clearly suggest that more fundamental research is needed in this area to identify the nature of dendritic interiors.
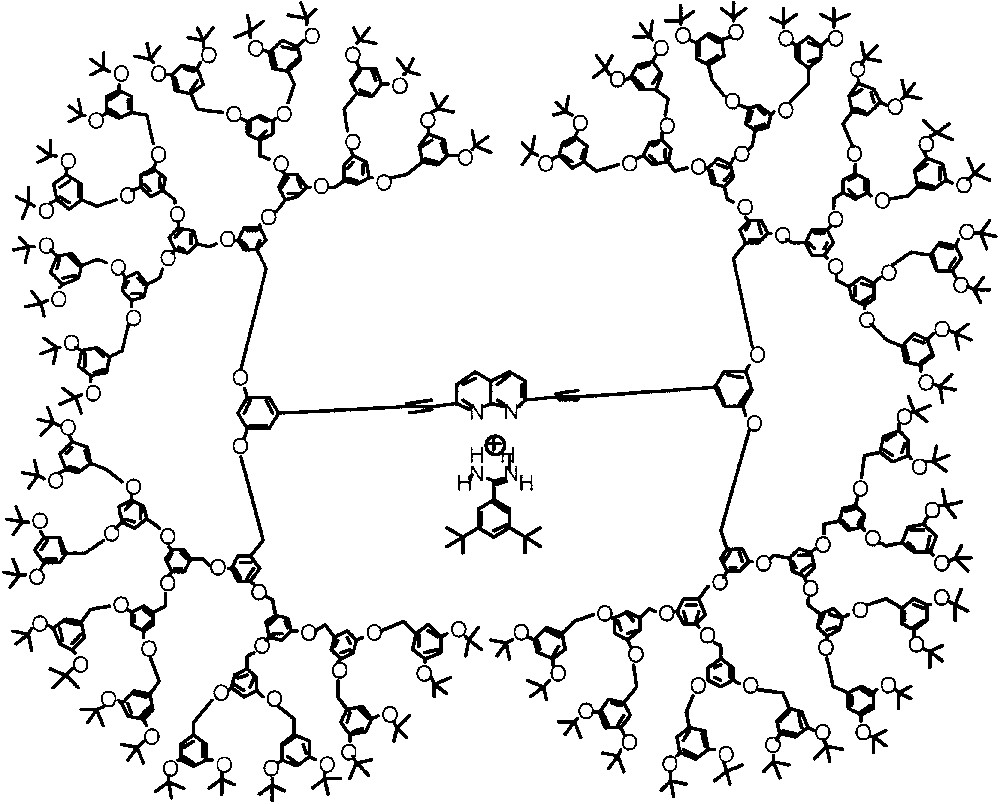
Benzyl ether dendrimer with naphtyridine host at the core, which is capable of binding to a benzamidinium guest molecule through hydrogen bonding.
3 Steric protection of functionalities
Encapsulation is the simplest form of mimicking the functions of nature’s macromolecular architectures with dendrimers. Functionalities that are found in nature, as well as other artificial functionalities have been encapsulated using dendrimers. Examples include metal complexes of bipyridine [8], tripyridine [9], oxazolines [10], porphyrins [11], and phthalocyanins [12], as well as other photoactive, electroactive or catalytically active functionalities such as ferrocene [13], iron-sulfide clusters [14], azobenzene [15], pyrene [16], fullerenes [17], nitroxide initiators [18], lanthanide ions [19], conjugated and non-conjugated polymers [20]. A few illustrative examples are outlined here.
Newkome and co-workers investigated the interaction between two dendritic components of a bis(terpyridine)ruthenium(II) complex [9]. The ‘lock-and-key’ interaction between the two components was monitored by cyclic voltammetry. The combination of second-generation dendrons for both the ‘lock’ and the ‘key’ exhibited reversible redox peak in the CV. However, the interaction between a fourth-generation ‘lock’ and the second-generation ‘key’ resulted in an irreversible redox behavior. These results suggest that the ruthenium core is efficiently shielded in the fourth generation dendron by steric protection, as illustrated in Fig. 4. Similar experiments with interaction between dendrons and dendrimers were also performed by others [11b].

Dendrons possessing terpyridine groups at the core and formation of ruthenium complexes in a ‘lock-and-key’ fashion is illustrated. Circles with increasing sizes denote increase in the number of generations.
Another example of steric protection by dendrimers involves the shielding of a porphyrin oxidation catalyst reported by the Moore and Suslick groups [21]. In this case, a manganese porphyrin complex was attached to phenylester dendrons as shown in Fig. 5. This complex exhibits excellent oxidative stability due to efficient shielding. Treatment of this complex with iodosobenzene affords a manganese-oxo complex, which can be used as an epoxidation catalyst. Interestingly, the catalyst based on the second-generation dendrimer exhibited a size-selective epoxidation of olefins. The terminal olefins were oxidized selectively compared to internal olefins. This selectivity is up to four times higher than the analogous manganese-oxo porphyrin complex without the appended dendrons.
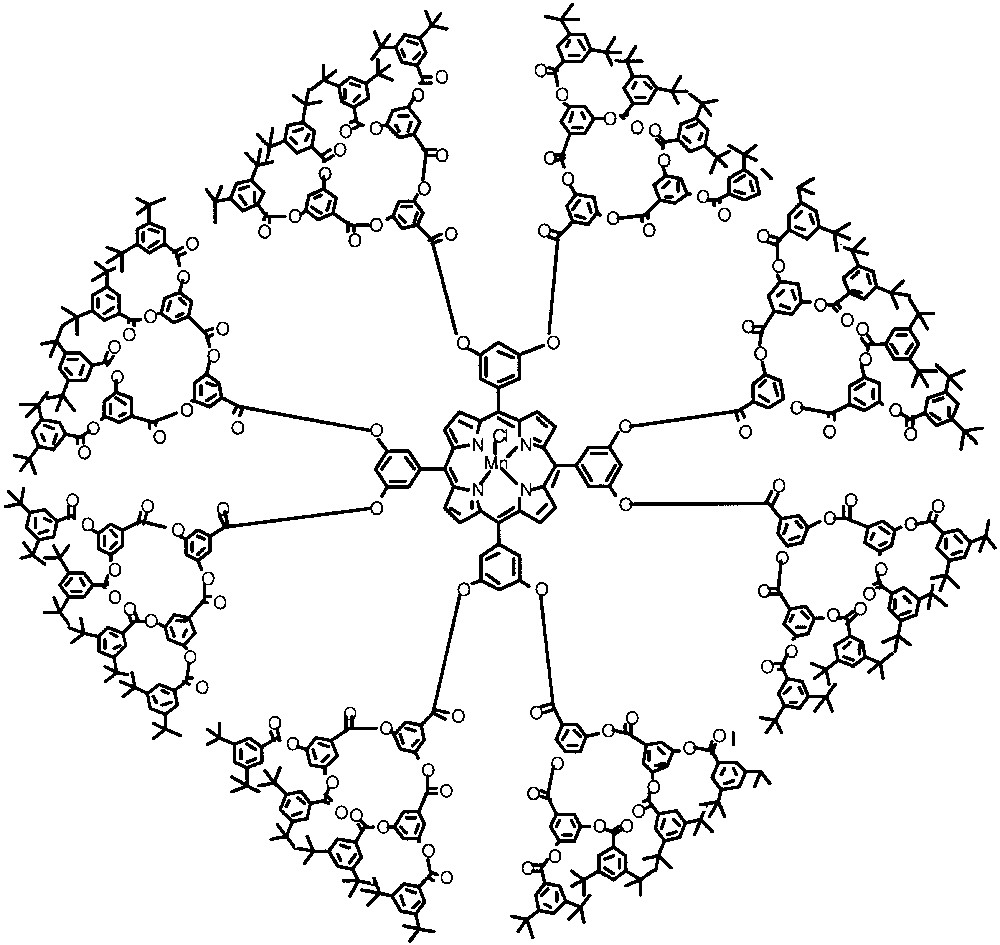
Poly(phenylester) dendrimer with manganese porphyrin catalyst at the core.
Another form of steric protection involves site-isolation of redox active units, a theme that is also prevalent in biological structures. For example, metalloporphyrins encapsulated in the interiors of proteins such as cytochrome c and ferrodoxin exhibit very high redox potentials. Diederich and co-workers systematically changed the nature of the dendrimer backbone encapsulating the redox active unit in order to identify the role of the encapsulating material on redox potentials. In a water-soluble dendrimer system, the Diederich group observed a redox potential that is about 0.42 eV higher than those observed without dendritic shielding (Fig. 6) [22]. Interestingly, the difference in oxidation potential between the dendrimer generations was negligible in dichloromethane. In a more recent paper, Diederich and co-workers measured the redox potentials of a similar model system in three different solvents (Fig. 7) [23]. In this system, the redox potential of the second-generation dendrimer was independent of the solvent, while the porphyrin control molecule and the more solvent exposed first generation dendrimer exhibited solvent-dependent redox potentials. These observations were attributed to the encapsulation of the metalloporphyrin core from the solvent environment in the second generation. Similar studies on dendrimers with appended metal-porphyrin and related complexes for catalysis and for investigating the possible biomimetic encapsulation of electroactive units were reported [8–20,24].
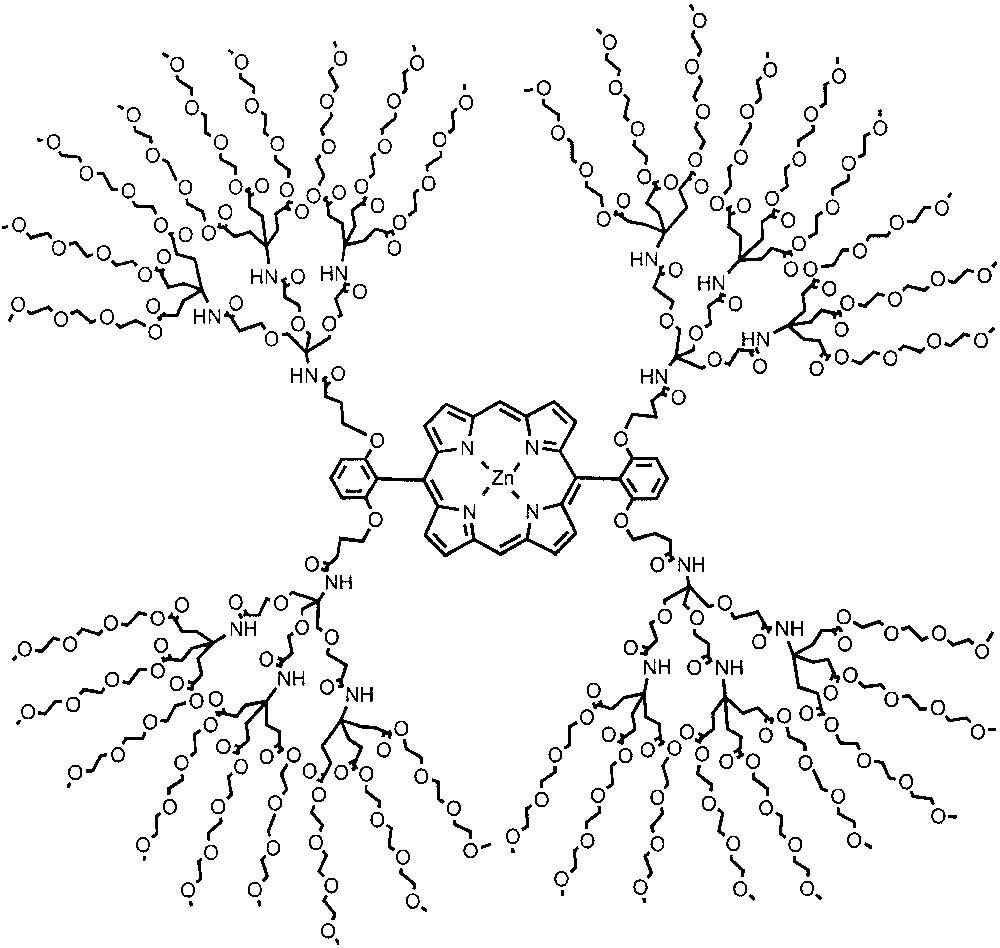
Water-soluble dendritically encapsulated zinc–porphyrin complex.
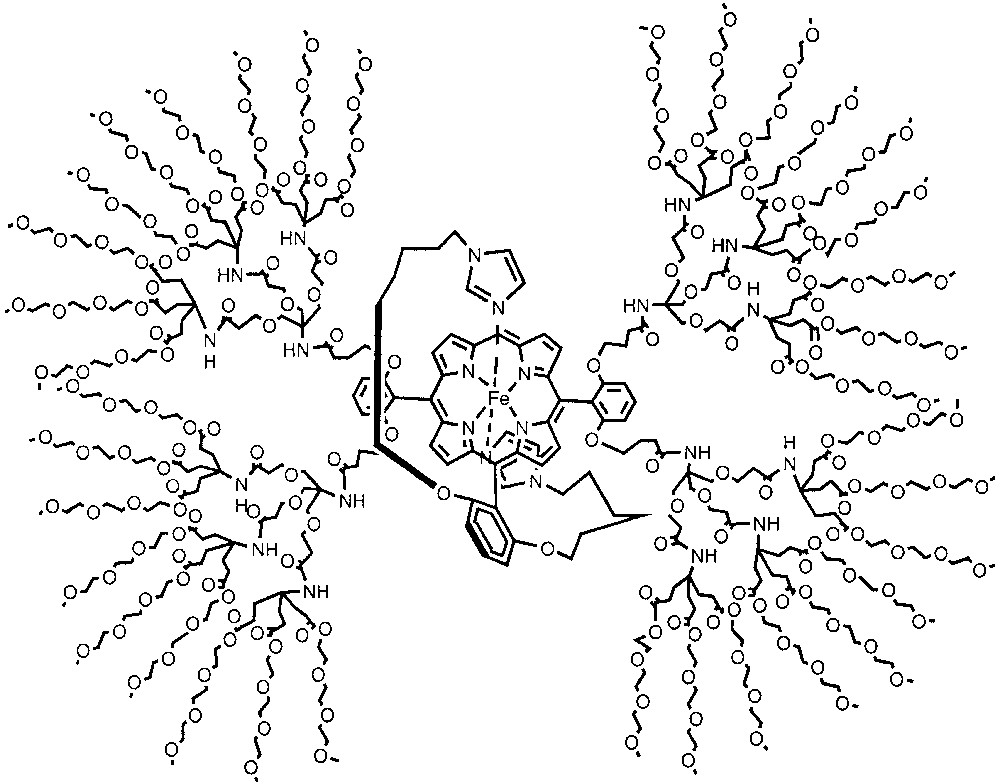
Dendritic mimic of cytochrome.
4 Functionalization of dendritic interior
An efficient way of achieving a microenvironment in the dendritic core that is different from the solvent is to build amphiphilic dendrimers. In 1991, Newkome and co-workers reported a poly(ammonium carboxylate) amphiphilic dendrimer shown in Fig. 8, which contained a hydrocarbon interior and a carboxylate periphery with ammonium counter ions [25]. These molecules were appropriately called ‘unimolecular micelles’. The micellar character of this dendrimer was established by incorporation of hydrophobic guest molecules such as phenol blue, naphthalene, and diphenylhexatriene. The presence of these molecules in the interior was confirmed by UV–visible spectroscopy and by fluorescence lifetime decay experiments. Similarly, amphiphilicity and globular shape of dendrimers have been combined by other research groups to bring about useful properties [26].
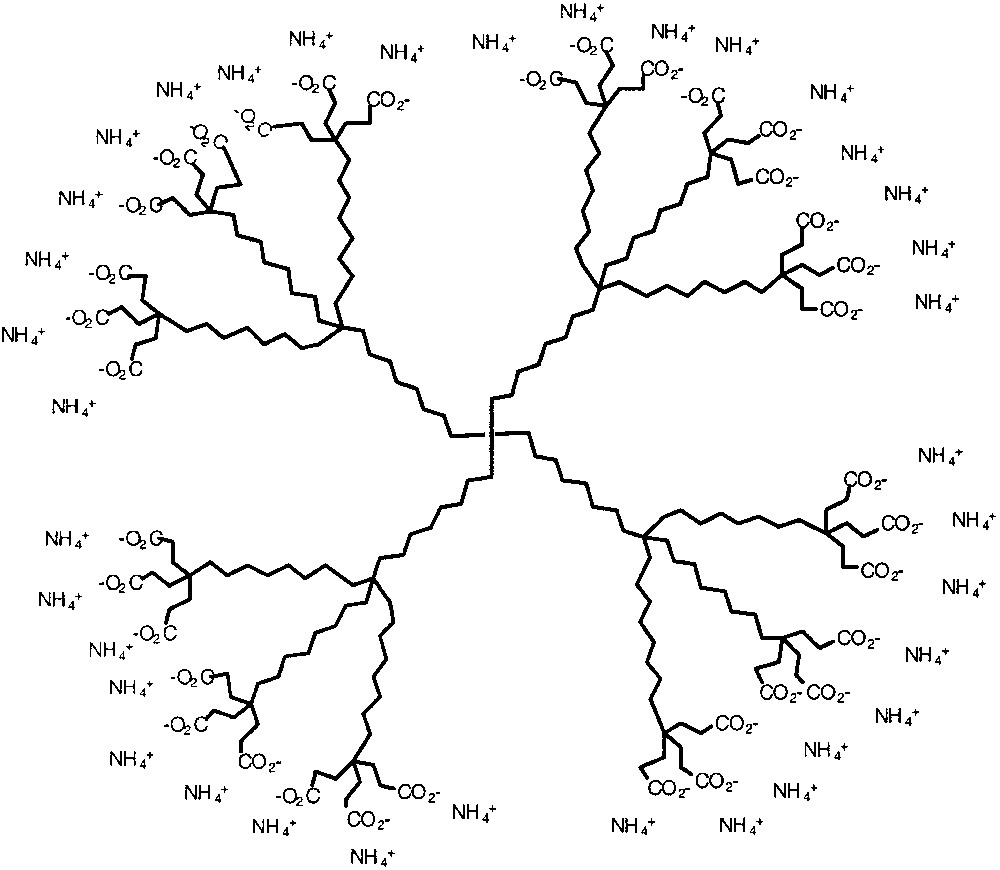
Unimolecular dendritic micelle with hydrocarbon interior and carboxylate periphery.
In all the dendrimers referred to in the last paragraph, the amphiphilicity arises from the difference in polarity between the dendrimer backbone and the functional groups attached to the periphery. However, in proteins, almost all the side chains of hydrophobic amino acids are directed towards the interiors. Similarly, the hydrophilic amino acid side chains are directed towards the exterior of these proteins with significant consistency. These biomacromolecules can therefore be considered as unimolecular micelles. However, an important structural requirement that will further enhance dendrimers’ repertoire in this arena involves the ability to selectively functionalize the concave interiors of these macromolecules, i.e. selectively direct functional groups towards the interior of the globular dendrimer. A flexible backbone is a pre-requisite to obtain globular shape at high generations. Attaining conformational control over the orientation of the functional groups is non-trivial, when the molecules are built with flexible linkers. A new approach has been introduced in order to achieve control over functional group orientation by rendering the macromolecules amphiphilic [27]. In this design, the dendrimers are uniformly amphiphilic over the entire globular surface: when the convex face of the dendrimer is hydrophilic, the concave face will be hydrophobic and vice versa. The nature of the functional groups directed towards the concave interior of the dendrimer will be driven by solvophobic interactions. In this design, the inherent flexibility of the dendritic backbone can be expected to yield the different solvent dependent conformations A and B, as shown in Fig. 9.

The possible conformations (A and B) of the amphiphilic dendrimer in polar and apolar solvents, respectively.
The monomer design involves a unit that has the AB2 functional groups for the dendrimer growth and the amphiphilic functional groups in orthogonal planes (Fig. 10). The amphiphilic substituents are placed on opposite sides of the plane containing the AB2 moieties. Such relative placement of the functional groups dictates that the amphiphilic moieties are in a plane perpendicular to that of the macromolecular backbone upon assembly of the dendrimer. The geometric arrangement of the dendrimer also dictates that the hydrophobic and the hydrophilic functionalities are in opposite faces of the globular dendrimer; and thus the structures A and B should result (Figs. 9 and 10). A monomer unit that satisfies these structural requirements is represented by the biphenyl molecule in Fig. 10, in which the hydrophilic unit is a triethyleneglycol monomethyl ether (TEG) moiety and the hydrophobic unit is an n-butyl group. Synthesis of dendrons up to the fourth generation has been reported (Fig. 11). However, solubility problems hampered the studies that would investigate the structural hypothesis proposed. Experiments are underway to modify the functional groups and render the dendrimers soluble in both polar and apolar solvents.
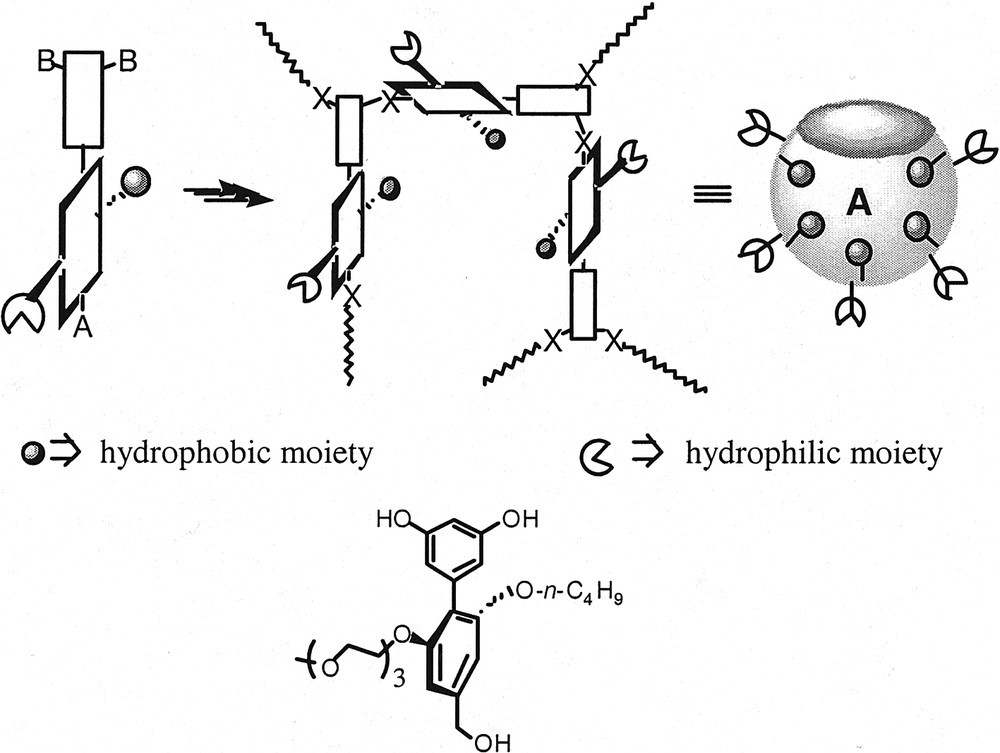
Cartoon of the AB2 monomer with the amphiphilic groups on the orthogonal plane; the dendrimer growth and its conformation in a hydrophilic solvent is represented.
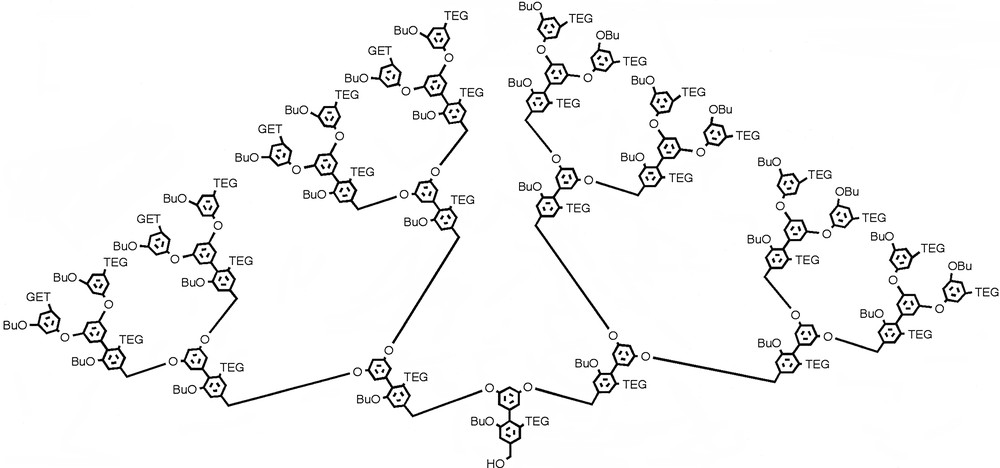
Fourth-generation dendron based on the biaryl system. Butyl and triethylene-glycol-monomethyl ether are the hydrophobic and hydrophilic functionalities respectively.
5 Functional group diversity in dendrimers
In addition to directing functional groups towards the concave interiors of the dendrimer, another step towards making these macromolecules more versatile as biomimetic structures involves the ability to achieve diversity in functional group presentations. In order to achieve this goal, synthetic strategies should be available, in which each monomer unit within a dendrimer can be different. Both divergent and convergent synthetic strategies have been used for functional group diversity in dendrimers. While the divergent approach allows for the incorporation of several monomers in a single layer of the dendrimer, the inherent nature of the strategy would result in an uncontrolled incorporation of various functionalities [28]. For the purpose of biomimetics, it is desirable that the spatial placement of monomer units, i.e. the sequence, is precisely controlled. The convergent approach is useful for this purpose [29]. Since dendrimer synthesis involves repetitive steps, it is easy to imagine varying monomer units in each layer of the dendrimer. However, this involves the ability to differentiate the two B functionalities in an AB2 monomer unit. Stoichiometric control of reagents to obtain and isolate mono-substituted products in the conventional convergent approach constitutes one route to achieving functional group diversity [30]. However, separation of the mono-substituted product from a statistical mixture of products can be both wasteful and time consuming. Three different methodologies have been developed over the past few years that will allow for sequencing dendrimers.
In one approach, Thayumanavan and co-workers used an AB2 monomer unit in which one of the B units is protected [31]. This approach was demonstrated by the mono protection of one of the phenolic groups with an allyl moiety in 3,5-dihydroxybenzyl alcohol. The resultant monomer was treated with one alkylating agent to cleanly afford the mono-substituted compound. The mono-substituted product was then treated with sodium borohydride in the presence of a palladium catalyst to afford the deprotection of the allyl group. The resultant phenol was then treated with a second electrophile to afford the differentially substituted G–1 dendron. Conversion of the hydroxymethyl moiety to bromomethyl group and iteration of the above mentioned steps resulted in the synthesis of a G–3 dendron with eight different peripheral functionalities. The synthetic scheme is shown in Fig. 12. Note that the synthesis of a dendrimer in which each of the peripheral monomers is different, is an indirect demonstration of the ability to vary each of the monomer units within the dendrimer.
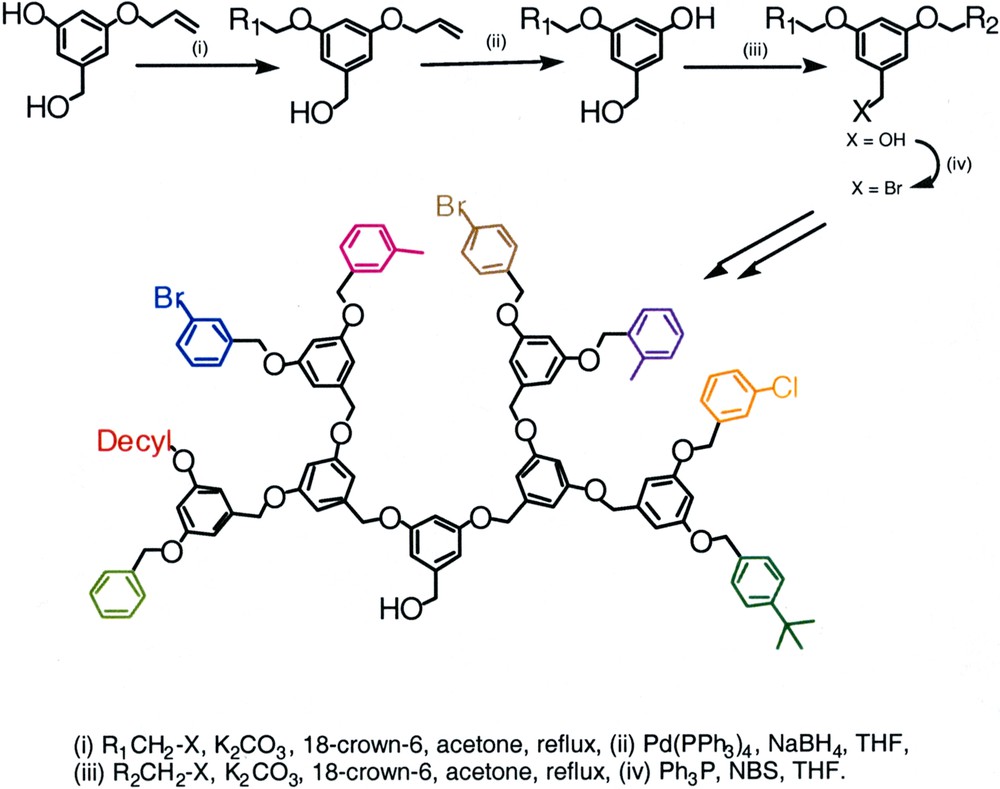
Layout of the synthesis of the third-generation dendron using protection–deprotection methodology.
Another strategy involves the incorporation of three different molecules to a common core unit, where Simanek and co-workers utilize the differential reactivities of di–, mono–, and unsubstituted cyanuric chloride [32]. Reaction of cyanuric chloride with the first amino moiety can be achieved at 0 °C, whereas the second amino-substitution on to the triazine unit requires ambient temperature conditions; and introduction of the third substituent requires elevated temperatures. The synthetic approach is shown in Fig. 13. Using this approach, dendrimers that display one or two boc-protected amines on their periphery in a controlled manner were achieved by Simanek and co-workers.

Sequential incorporation of different amino groups onto the triazine moiety. Also, the triazine-based dendrimer with two different peripheral groups is given.
A third method that is complementary to the two strategies was introduced by Thayumanavan and co-workers [33]. In this approach, an ABB' monomer was used as the building block unit. In this monomer, under a certain condition the functionality B reacts with A selectively, while A can react with B′ under a different set of conditions. The repeat unit used for this purpose was ethyl 3-hydroxy-5-hydroxymethylbenzoate. Here B is the phenol, B′ is the primary alcohol, and the masked form of A is the ester group. Treatment of the monomer unit with one equivalent of an electrophile in the presence of potassium carbonate and 18-crown-6 in THF afforded the phenol substituted product. Addition of sodium hydride and the second electrophile to the same reaction pot affords the incorporation of the second electrophile on to the hydroxymethyl moiety. Reduction of the ester to a hydroxymethyl group followed by conversion to a bromomethyl functionality makes the resultant G–1 dendron an electrophile for building the next generation dendron. The versatility of this synthetic methodology was demonstrated by synthesizing a third generation dendron with six different peripheral functionalities (Fig. 14).

Schematic representation of the synthesis of a third-generation dendron with rix different peripheral functionalities.
6 Summary
Dendrimers clearly have the potential to be macromolecular biomimetic materials. Due to the ability in achieving control over size, shape, and sequence, dendrimers provide a unique opportunity in this arena. From a functional point of view, dendrimers have already demonstrated its potential through several examples in the steric protection of photoactive, electroactive, and catalytically active functionalities, size-selectivity in substrates for catalytic activity, etc. From a structural detail standpoint, efforts have started to push the frontier to render dendrimers into more versatile biomimetic architectures. Mimicking structures in nature, such as proteins, with artificial macromolecular architectures is certainly attractive. Combining the strategies for selectively directing functional groups along with sequencing the dendrimer is one of the obvious next steps in our own research group.
Acknowledgements
This work in our laboratory is supported by the NIGMS of the National Institutes of Health (R01 GM065255-01) and NSF-Career award (NSF-CHE-0134972). Support through a Cottrell Scholar award of the Research Corporation is also acknowledged. We are indebted to all the co-workers from Tulane University and the University of Massachusetts, who have contributed to the work described in this account.