1 Introduction
In nature, biological organisms produce polymer-inorganic hybrid materials such as bone, teeth, diatoms, and shells. These hybrids have superior mechanical properties as compared to synthetic hybrids. For example, the abalone shell, a composite of calcium carbonate with a few percent of organic component (Fig. 1), is 3000 times more fracture resistance than a single crystal of the pure mineral [1]. The core of the organic template is composed of a layer of β-chitin, layered between ‘silk-like’ glycine- and alanine-rich proteins. The outer surfaces of the template are coated with hydrophilic acidic macromolecules. Natural inorganic-organic hybrid materials are formed through mineralization of inorganic materials on self-assembled organic materials. Biopolymers and low-molecular-weight organic molecules are organized into nanostructures, and used as flameworks for specifically oriented, shaped inorganic crystals such as calcium carbonate, hydroxyapatite, iron oxide, and silica. These processes are using an aqueous solution at a temperature below 100 °C. Moreover, no toxic intermediates are produced in these systems. Therefore, the processes and materials that control such crystal growth are of great interest to material scientists who seek to make composite materials and crystalline forms analogous to those produced by nature.

Schematic illustration of the nacre of abalone shell.
In these mineralized tissues, crystal morphology, size, and orientation are determined by local conditions and, in particular, the presence of ‘matrix’ proteins or other macromolecules [2]. Morphological control can also be accomplished by adsorption of soluble additives onto specific faces of growing crystals, altering the relative growth rates of the different crystallographic faces and leading to different crystal habits. Since these processes take place usually at an organic-inorganic interface, the organic portion providing the initial structural information for the inorganic part to nucleate on and grow outwards in the desired manner. Most researchers’ interest lies in understanding how organized inorganic materials with complex morphological forms can be produced by biomineralization processes, and how such complexity can be reproducibly synthesized in biomimetic systems. Due to the complexity of the natural biomineralization systems, mineralization research has been studied on model organic interfaces [3–8]. Various types of organic matrices for use as structural templates for crystallization of inorganic materials should be easily designed and synthesized.
Calcium carbonate makes an attractive model mineral for studies in the laboratory, since its crystals are easily characterized and the morphology of CaCO3 has been the subject of control in biomineralization processes. The precipitation of calcium carbonate in aqueous solution is also of great interest for industrial and technological applications. The particular interest in this system is due to the polymorphism of calcium carbonate, which has three anhydrous crystalline forms, i.e., vaterite, aragonite, and calcite, in order of decreasing solubility and increasing stability. The three polymorphs have markedly different physicochemical characteristics, and it is often found that less stable forms are stabilized kinetically. Vaterite transforms into the thermodynamically most stable calcite via a solvent-mediated process [9]. Organizations of calcium carbonate crystals in biological systems in the three polymorphs, calcite, vaterite and aragonite, are well known illustrations of the biomineralization processes. Calcite and aragonite are widespread in marine organisms, and vaterite, monohydrocalcite, and amorphous calcium carbonate are formed and stabilized by some organisms [10]. The manner in which organisms control polymorph formation is not well understood.
2 Why are dendrimers used for calcium carbonate crystallization?
An in vitro study of biomineralization provides useful information for the design of organic templates. Falini and co-workers assembled in vitro a complex containing the major matrix components present in a mollusk shell, namely β-chitin, a silk-fibroin-like protein, and water-soluble acidic macromolecules [11]. When this assemblage was placed in a saturated solution of calcium carbonate, multi-crystalline spherulites formed within the complex. They extracted aspartic-acid-rich glycoproteins from an aragonitic mollusk shell layer or a calcitic layer. These were aragonite if the added macromolecules were from an aragonitic shell layer, or calcite if they were derived from a calcitic shell layer. In the absence of the acidic glycoproteins, no mineral formed within the complex. Since the high-dimensional structures of these proteins were unknown, the detail mechanism of the effect of nucleation at the molecular level remains unknown. Despite that, these in vitro study motivate us to design artificial templates for controlled nucleation of minerals.
Model systems, in which low-molecular-weight organic additives are used to study the effect of molecular properties such as charge and functionality on inorganic crystallization, are providing insights into the possible mechanisms operating in biology. These additives were chosen to mimic the active protein ligands. The influence of organic molecules on the nucleation and crystal growth of calcium carbonate has been studied by a number of authors [12–16]. Because the proteins that have been found to be associated with biominerals are usually highly acidic macromolecules, simple water-soluble polyelectrolytes, such as the sodium salts of poly(aspartic acid) and poly(glutamic acid), were examined for the model of biomineralization in aqueous solution. Studies of inorganic crystallization in the presence of soluble polymers, modeled on biogenic proteins, have shown that selectivity for certain crystal faces appears to be highly dependent on the secondary structure of the macromolecules. For example, poly-l-aspartate, with a predominantly β-sheet conformation, produces more aragonite than poly-l-glutamate, which has a random conformation [17]. Crystallization of CaCO3 in the presence of various synthetic non-peptide polymers has been investigated as a model of biomineralization [7, 8].
For such synthetic linear polymers, it has been difficult to unambiguously assign a structure-function relationship in the context of their activity in crystallization assays, since they mostly occur in the random-coil conformation. Dendrimers are monodisperse macromolecules with a regular and highly branched three-dimensional architecture. As shown in Fig. 2, the starburst structures are disk-like shapes in early generations, whereas the surface branch cell becomes substantially more rigid and the structures are spheres [18]. Due to unique and well-defined secondary structures of the dendrimers, the starburst dendrimers should be a good candidate for studying inorganic crystallization.

Poly(amidoamine) (PAMAM) dendrimers with carboxylate groups at the external surface.
Poly(amidoamine) (PAMAM) dendrimers with carboxylate groups at the external surface, termed half-generation or G = n.5 dendrimers, have been proposed as mimics of anionic micelles or proteins [19]. Later than 4-generation PAMAM dendrimers are nearly spherical in shape according to molecular simulations [20]. The half-generation PAMAM dendrimers using various photophysical probes indicate a structural surface transition in the dendrimer appearance on going from generation 3.5 to 4.5 [21]. The morphology of the early generation (0.5–3.5) dendrimers is an open structure, and the internal tertiary amines and amide groups are available for bonding. The later generations have more spheroidal and close-packed surface structures.
Another example of dendrimers used for precipitation of CaCO3 was reported by Summerdijk et al. They used poly(propyleneimine) dendrimers, modified with long aliphatic chains, to give a new class of amphiphiles that display a variety of aggregation states due to their conformational flexibility [22]. In the presence of octadecylamine, poly(propyleneimine) dendrimers, modified with long alkyl chains, self-assemble to form remarkably rigid and well-defined aggregates. When the aggregate dispersion was injected into a supersaturated solution of calcium carbonate, the aggregates stabilized the normally unstable amorphous calcium carbonate [23]. Over the course of four days a discrete population of rhombohedral calcite crystals was also identified, alongside large isolated amorphous calcium carbonate particles. This process might affect the gradual release of the aggregates. Although amorphous calcium carbonate has been observed as a short-lived intermediate in the presence of various scale prevention agents, only biological systems allow the coexistence of amorphous and crystalline calcium carbonate for extended periods of time. Stabilization of the amorphous phase against the transformation to the thermodynamically stable form results in the formation of a unique inorganic-organic hybrid material.
3 Crystallization of CaCO3 with the anionic PAMAM dendrimers
The following sections show our recent research on crystal nucleation and the growth of calcium carbonate in aqueous solution by poly(amidoamine) (PAMAM) dendrimers with carboxylate groups at the external surface. Since the crystallization of CaCO3 depends on nucleation condition, two different conditions were employed.
3.1 Crystallization by double-jet method
The precipitations of CaCO3 in the presence and the absence of the G1.5 PAMAM dendrimer were carried out in a double jet reactor [24, 25] to prevent heterogeneous nucleation at the glass walls, as shown in Fig. 3. The two reactants (CaCl2 and Na2CO3) are injected via capillaries into the reaction vessel under vigorous stirring to prevent heterogeneous nucleation at the glass wall. The two capillary ends are joined together so that a high local reactant concentration and thus extreme supersaturation is achieved at the moment when the two reactants leave the capillaries, which provides an immediate nucleation of CaCO3. The nuclei are then immediately transported to regions of lower CaCO3 concentration and can grow further. The CaCO3 crystal formation occurring after an excess addition of reactants was easily observed as a sudden increase in the turbidity of the solution. A crystallization of CaCO3 in the presence of the PAMAM dendrimers with carboxylate groups at the external surface resulted in the formation of spherical vaterite crystals (Fig. 4a), whereas rhombohedral calcite crystal was formed without the additive [26, 27]. In the presence of the dendrimers, further washing of the vaterite crystal with water did not change the crystal morphology.
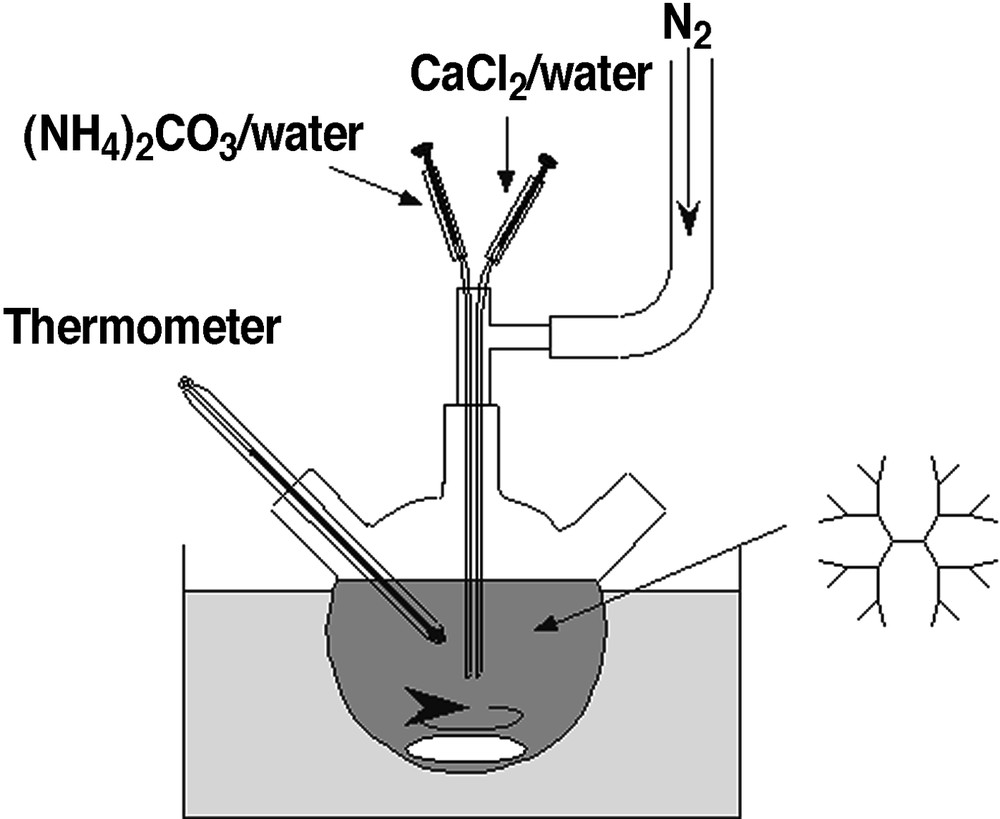
The experimental set-up of a double jet reactor for the precipitation of calcium carbonate.

Scanning electron micrographs of the crystalline products in the presence of (a) G1.5 and (b) G3.5 PAMA dendrimers.
The precipitation of CaCO3 in the presence of Na-salt of poly(acrylic acid) (PAA) (Mn = 5100) was also carried out under the same conditions described above. In the presence of the Na-salt of poly(acrylic acid) (PAA), the formation of crystalline CaCO3 was prevented. The crystalline CaCO3 was hardly collected after incubation at 25 °C under N2 for four days. This indicates that PAA acts as an inhibitor for crystal formation.
Vaterite transforms easily and irreversibly into thermodynamically more stable forms when in contact with water. The complete phase transformation into the thermodynamically stable calcite occurs within three days, usually much faster under the conditions described above [25]. It is well known that vaterite transforms into stable calcite via a solvent-mediated process [28]. Under the precipitation condition, extreme supersaturation is achieved at the time and provides an immediate nucleation of CaCO3, which is not affected by the organic additives. If the Ca–O bonds of the polymer ligand are easily dissociated by water, the polymer is thought not to be occluded in the CaCO3 crystal. Then the solvent-mediated vaterite–calcite transformation might be performed. Strong Ca–O bonds are thus required to control the polymorph of CaCO3. In the presence of the dendrimers, the vaterite crystal with incubation in water for one week did not change the crystal morphology. This indicates that the vaterite surface was stabilized by the carboxylate-terminated PAMAM dendrimer to prevent the phase transformation to calcite in an aqueous solution.
The complexes of anionic dendrimers with calcium ions are considerably stronger for higher generations than for lower generations. The particle sizes of the spherical vaterite crystals obtained in the presence of the PAMAM dendrimers were dependent on the generation numbers and concentration of the PAMAM dendrimers (Table 1) [27]. As the generation number of the PAMAM dendrimer increased from G1.5 to G3.5, the particle size of the spherical vaterite was decreased from 5.5 ± 1.1 to 2.3 ± 0.7 μm (Fig. 4b). On a further increase in the generation number to G4.5, the particle size was not changed (2.3 ± 0.8 μm). At the lower concentration of the G1.5 PAMAM dendrimer, corresponding to 0.13 mM of –COONa, the particle size of the vaterite crystals was 5.6 ± 1.4 μm. As the concentration of –COONa increased from 0.26 to 8.33 mM, the particle sizes of the spherical vaterite were reduced from 5.5 ± 1.1 to 2.5 ± 0.6 μm. In the case of the G3.5 PAMAM dendrimer, the concentration of –COONa increased from 0.13 to 0.26 mM, the particle size was also decreased from 5.8 ± 1.8 to 2.3 ± 1.8 μm. With a further increase of the concentration to 8.33 mM, the particle size was decreased to 1.5 ± 0.6 μm. The particle sizes were larger for the lower generation (G1.5) than for the higher generation (G3.5) at any concentration of the PAMAM dendrimers.
The particle sizes of vaterite in the presence of PAMAM dendrimers
[–COONa] (mM) | Generation of PAMAM dendrimer | Particle sizea (μm) |
0.13 | G = 1.5 | 5.6 ± 1.4 |
0.26 | G = 1.5 | 5.5 ± 1.1 |
8.33 | G = 1.5 | 2.5 ± 0.6 |
0.13 | G = 3.5 | 5.8 ± 1.8 |
0.26 | G = 3.5 | 2.3 ± 0.7 |
8.33 | G = 3.5 | 1.5 ± 0.6 |
0.26 | G = 4.5 | 2.3 ± 0.8 |
The yields of the crystalline products in the presence of the G1.5 and G3.5 dendrimers were 61 and 30%, respectively. Due to the complexation of the dendrimer with calcium ions in aqueous solutions, the saturated concentration of calcium ions in the presence of the G1.5 and G3.5 PAMAM dendrimers were 1.3 and 2.8 times higher than that in the absence of the additive. This result indicates that the higher generation of the dendrimer acts as an inhibitor for crystal formation. The inner cores of the PAMAM dendrimers are hydrophilic and potentially open to small hydrophilic molecules, since interior nitrogen moieties serve as complexation sites. The amount of calcium ions on the anionic dendrimers is considerably higher for the higher generations than for the lower generations.
The PAMAM dendrimer contents in the crystalline CaCO3 in the presence of the G1.5 PAMAM dendrimer and the G3.5 PAMAM dendrimer at the same concentration (corresponding to 8.33 mM of –COONa) were 27 and 39 wt%, respectively. These data suggested that an adsorption of the G3.5 PAMAM dendrimer on the vaterite surface was higher than that of the G1.5 PAMAM dendrimer. The spherical vaterite crystals were aggregates of vaterite nanoparticles with diameter of 10 to 30 nm [25]. Formation of the vaterite particles involves two processes, i.e. the nucleation of vaterite nanocrystals and the aggregation of the nanocrystals. The nanoparticle surface might be covered with the PAMAM dendrimer. The complexation ability of the higher generation of the PAMAM dendrimer is stronger than that of the lower generation of the PAMAM dendrimer [20]. Aggregation of the nanoparticles in the presence of the higher generation of the G3.5 PAMAM dendrimer might be prevented compared to that in the presence of the lower generation of the G1.5 PAMAM dendrimer.
Vaterite is thermodynamically the most unstable of the three crystal structures. Vaterite, however, is expected to be used in various purposes, because it has some features such as high specific surface area, high solubility, high dispersion, and small specific gravity compared with the other two crystal systems. Spherical vaterite crystals have already been reported in the presence of divalent cations [29], a surfactant [bis(2–ethylhexyl)sodium sulfate (AOT)] [28], poly(styrenesulfonate) [30], poly(vinyl alcohol) [12], and double-hydrophilic block copolymers [25]. The control of the particle size of spherical vaterite should be important for application as pigments, fillers and dentifrice.
3.2 Crystallization by carbonate diffusion method
Crystallization of CaCO3 highly depends on the nucleation condition. The precipitation of CaCO3 in the absence or the presence of the G4.5 PAMAM dendrimer was carried out by a ‘carbonate diffusion method’ [30], similar to the method described by Addadi et al. [31]. A solution of the dendrimer with calcium chloride in 200 ml of distilled water was adjusted to pH 8.5 with aqueous NH3, and then placed in a closed desiccator containing crushed ammonium carbonate (Fig. 5). Carbon dioxide was introduced to the solution via vapor diffusion. The critical point of the appearance in the turbidity of the solution was observed at around 5 min. These solutions were kept at 30 °C under N2 for 1 day. The crystalline CaCO3 was collected and washed with water several times to remove contaminated dendrimers that were not involved in the crystal. Formation of the crystalline CaCO3 at different feed ratio of the G4.5 PAMAM dendrimer to calcium ions was studied with the constant concentration of calcium ions at 0.1 M. The results are summarized in Table 2 [32]. At the low concentration of the G4.5 PAMAM dendrimer (runs 2 and 3), calcite was predominantly formed. However, as the concentration of –COONa increased to 5.3 mM, vaterite formation appeared strongly. Further increase of the concentration of –COONa to 10.6 mM also formed vaterite. Although calcite was predominantly formed at the concentration of the G4.5 PAMAM dendrimer corresponding to 2.65 mM of –COONa (run 3), the crystal phase of the obtained CaCO3 at the higher concentration of the G4.5 PAMAM dendrimer at 5.3 mM consisted entirely of vaterite (98% by IR). These results indicate that the presence of the dendrimer affected polymorphs of CaCO3 crystallization.

The experimental set-up of a carbonate diffusion method for the precipitation of calcium carbonate.
The precipitation of CaCO3 in the absence and the presence of the G4.5 PAMAM dendrimer (adapted from [30])
Run | [–COONa] (mM) | [Ca2+] (M) | [–COONa]/[Ca2+] | Polymorphsa | Vaterite contentb (%) |
1 | 0 | 0.1 | 0 | calcite + vaterite | 56 |
2 | 0.53 | 0.1 | 0.0053 | calcite >> vaterite | 15 |
3 | 2.65 | 0.1 | 0.027 | calcite | 0 |
4 | 5.3 | 0.1 | 0.053 | vaterite >>> calcite | 98 |
5 | 10.6 | 0.1 | 0.11 | vaterite >>> calcite | 93 |
6 | 0 | 0.05 | 0 | calcite + vaterite | 70 |
7 | 0.53 | 0.05 | 0.011 | calcite | 0 |
8 | 1.33 | 0.05 | 0.027 | calcite >>> vaterite | 13 |
9 | 2.65 | 0.05 | 0.053 | vaterite >>> calcite | 89 |
10 | 5.3 | 0.05 | 0.11 | vaterite >>> calcite | 93 |
The precipitation of CaCO3 in the absence of any additives was carried out under the same nucleation condition (run 1 in Table 2). The crystal phase of the obtained CaCO3 was a mixture of calcite and vaterite by IR. The vaterite content was slightly higher than the calcite content. However, calcite was predominantly formed at the low concentration of the G4.5 PAMAM dendrimer corresponding to 0.53 and 2.65 mM of –COONa (runs 2 and 3). Due to the complexation of the PAMAM dendrimer with calcium ions in aqueous solutions, crystallization of calcium carbonate in the presence of the dendrimer is inhibited compared with that in the absence of the additive with constant concentration of calcium ions [27]. The interior nitrogen moieties of the dendrimers serve as complexation sites. According to the literature, relatively high supersaturations in high pH values favor the precipitation of vaterite [33]. The presence of the dendrimer decreases the concentration of free calcium ions [27, 32], which were not bound to the dendrimer, resulting in the higher calcite contents compared with that in the absence of the dendrimer.
SEM observations showed that the most crystals obtained in the absence and the low concentration of the G4.5 PAMAM dendrimer were rhombohedral. In the high concentration of the G4.5 PAMAM dendrimer, the vaterite products were spherical. Each shape of CaCO3 is a typical morphology for each polymorph. The particle sizes of the spherical vaterite particles obtained in the presence of the PAMAM dendrimer were depended on the concentration of the dendrimer. As the concentration of –COONa increased from 5.3 to 10.6 mM, the particle sizes of the spherical vaterite particles were reduced from 8.7 ± 1.0 to 5.2 ± 3.0 μm.
The results of the precipitation of CaCO3 in the absence and the presence of the G4.5 PAMAM dendrimer at a lower concentration of calcium ion (0.05 M) are also summarized in Table 2. Although the vaterite content was higher than the calcite content in the absence of the dendrimer, calcite was predominantly formed at the concentration of the G4.5 PAMAM dendrimer corresponding to 0.53 and 1.33 mM of –COONa. As the concentration of –COONa increased to 2.65 mM, vaterite formation was strongly induced. At the higher concentration of calcium ions (0.1 M), the critical point of the morphology change from calcite to vaterite was observed on increasing concentration of –COONa increased from 2.65 to 5.3 mM. These results indicate that the feed ratio of –COONa and Ca2+ is a key factor for inducing vaterite formation.
The precipitations of CaCO3 in the presence of the G1.5, G3.5, and G4.5 PAMAM dendrimers were carried out with constant –COONa unit and calcium ions of 0.1 M. Although vaterite was predominantly formed by the G4.5 dendrimer, a relatively high amount of calcite was observed in the case of the G3.5 and G1.5 dendrimers (Table 3). These results suggest that the G4.5 dendrimer effectively induced vaterite formation compared with the earlier generation of the dendrimers. When the precipitations of CaCO3 in the presence of the G1.5, G3.5, and G4.5 PAMAM dendrimers were carried out by the double jet method as described above, in all cases, stable vaterite particles were obtained, in contrast to the present results. Under the previous precipitation condition, the extreme supersaturation is achieved and provides an immediate nucleation of CaCO3, which is not affected by the organic additives. The nuclei are then immediately transported to regions of lower CaCO3 concentration and can grow further. The spherical vaterite crystals were stabilized by the anionic PAMAM dendrimers in aqueous solution for more than 7 days. Vaterite transforms easily and irreversibly into thermodynamically more stable forms when in contact with water. These results suggest that the surface of the vaterite particles in the previous case was stabilized by the dendrimers to avoid water contact. On the other hand, the vaterite particles obtained by the carbonate diffusion method were transformed to calcite when the solution was incubated for 4 days. Although the dendrimer content in the vaterite particle was 7.27 wt% as determined by elemental analysis, these results indicate that the vaterite surface was not effectively stabilized by the PAMAM dendrimer. Under the present precipitation conditions, the dendrimers can play a role in initiating the nucleation of vaterite. Alternatively, the vaterite growth could be explained by a kinetic inhibition of the calcite nuclei by the dendrimers. Later than 4–generation of the PAMAM dendrimers are nearly spherical in shape according to molecular simulations [22]. The half-generation PAMAM dendrimers using various photophysical probes indicate a structural surface transition in the dendrimer appearance on going from generation 3.5 to 4.5 [20]. Complexation properties of the G4.5 dendrimers due to the stereochemical factor would be a major rolefor the vaterite mineralization.
The precipitation of CaCO3 in the presence of the G1.5, G3.5, and G4.5 PAMAM dendrimers (adapted from [32])
Run | Generation | [–COONa] (mM) | [Ca2+] (M) | [Ca2+]/[–COONa] | Polymorphsa | Vaterite contenta (%) |
1 | 1.5 | 5.3 | 0.1 | 0.053 | vaterite > calcite | 77 |
2 | 3.5 | 5.3 | 0.1 | 0.053 | vaterite + calcite | 48 |
3 | 4.5 | 5.3 | 0.1 | 0.053 | vaterite >>> calcite | 98 |
4 Conclusions
Over the past decade, considerable work have been performed on the mineralization of calcium carbonate in the presence of various low-molecular-weight organic molecules and polymers as templates and additives. These interesting efforts have led to fundamental developments in areas relating to the biomineralization process. However, there still remain many unknowns as to how natural organisms produce inorganic-organic hybrid materials. Since most synthetic polymers are not monodisperse molecules with random-coil conformations, the use of dendrimers allows assigning structure-function relationships in crystallization assays. Although the template mechanism of the dendrimers still seems to be of a complex nature because simultaneous CaCO3 nucleation and interaction with the polymer can be expected, the stereochemical factor is important for the affinity of the templates to manipulate the polymorph of CaCO3. We expect that the continuous cooperation of organic and polymer chemists with inorganic and biochemists is desirable to clarify the biomineralization process leading to the next industrial revolution.