1 Introduction
Combinatorial chemistry has successfully entered the field of materials and heterogeneous catalysis [1,2]. It is based on high-throughput experimentation, where libraries of potential catalysts are prepared and investigated in a parallel or an automated sequential manner to speed up development and discovery of materials with desired properties. Different approaches for the preparation and screening of libraries have been presented. A promising approach is the preparation of solid materials on libraries by liquid phase synthesis. Thereby, the use of pipetting robots is an easy way to create large libraries of different materials, which can be screened e.g for catalytic properties [3]. Screening of catalytic properties by high-throughput assays has been achieved in parallel and in fast sequential ways. One of the first parallelized methods used to analyse catalytic activity of library members in gas phase reactions is emissivity-corrected infrared thermography [4,5], which registers the heat of reaction on catalyst surfaces by infrared imaging. Infrared thermography is very sensitive and fast, but the method provides false positives with parallel or secondary reactions and is thus usually not suited for selective reactions [6]. Nevertheless, there are many reactions suitable for the study by infrared thermography, such as CO oxidation with air.
CO oxidation is a relatively simple reaction, irreversible at low temperatures without parallel or secondary reactions. CO oxidation is one of the basic model reactions reported upon in thousands of publications in surface science. It is most likely the best studied heterogeneously catalysed reaction on defined surfaces as well as on conventional catalysts. Nevertheless, there is still a need for better catalysts in a variety of practical applications. For low temperature fuel cells the final removal of residual CO in the hydrogen is of importance. It is presently achieved by oxidation with noble metal containing catalysts. These suffer under unselective oxidation and thus also reduce the hydrogen content. Highly active CO selective oxidation catalysts are required. In CO gas masks for fire fighters the presently used Hopcalite catalysts (a Mn–Cu mineral) deactivates in the presence of air humidity [7]. CO in air is also subject of concern in CO2 lasers [8]. In all these applications, CO-selective low temperature oxidation catalysts are required. Among the most active new catalysts for CO oxidation even far below room temperature are supported Au-catalysts discovered by Haruta et al. [9]. New noble metal free low temperature CO oxidation catalysts were investigated by parallel detection of reaction products with real-time photo acoustic measurements and Fe17Ni25Mn27Cu30 was found most active [10]. Several other materials such as NiOx [11], hydrous ruthenium dioxide [12], Co3O4 [13] and CuO-CeO2 [14] are known for CO oxidation at low temperatures.
There are already several studies on high throughput methods applied to CO oxidation catalysis in the literature. Various Au/Co3O4 and Au/TiO2 catalyst have been prepared with a dispensing robot and tested in a 16-well multiple-pass reactor under conditions close to conventional reaction conditions [15]. Cong et al. used RF sputtering to prepare three unique 120-member libraries of Rh/Pt/Cu, Rh/Pd/Cu, and Rh/Pt/Pd alloys, which were screened in a scanning mass spectrometer for CO oxidation at 350 °C [1]. Thereby, Rh/Cu (50/50 atom%) showed a promising catalytic activity. Recently, an inkjet-based polymerisable-complex method was used for the preparation of ternary libraries of oxide catalyst, based on the LaCoO3 system with substitutions of Sr and Ca on the lanthanum or A-sites and Cr on the cobalt or B-sites [16]. The activity for CO oxidation was screened above 150 °C by thermal infrared imaging and catalytic activity was confirmed in the LaxSryCozO3-δ and LaxCryCozO3-δ system. In the present work, we report the use of infrared thermography as high-throughput technique for the discovery and development of multi-component oxides for low temperature CO oxidation.
2 Experimental
2.1 Catalyst preparation
2.1.1 Catalyst libraries
The high-throughput synthesis of catalytic materials was based on versatile sol–gel recipes developed conventionally. The library design was carried out with the help of the software Plattenbau [17]. Plattenbau also generated the whole pipetting code (e.g. appropriate volumes of starting solutions for each oxide, pipetting sequence) for the automated preparation with a pipetting robot. Here, the pipetting robot Lissy from Zinsser Analytic was used. For the preparation of highly diverse metal oxides with Co, Mn and Ni as base oxides, up to 65 different liquids were positioned on the workspace of the robot. As precursors for the three base oxides Ni(II)- and Mn(II)-propionate dissolved in methanol (1.0 M) and Co(II)-propionate dissolved in ethanol (1.0 M) were used. As dopants 54 different elements solved in 2-propanol were used (0.1 M solutions for non-noble elements and 0.05 M for noble metals). Most of these doping compounds were nitrates or alkoxides. The pipetting of the precursor-solutions for the sol was performed in 2-ml GC vials, positioned in a rack with 50 positions. After shaking the mixed solutions for 1 h, the samples were dried for 5 days at room temperature (gel formation) before calcination in air at 300 °C for 5 h. Thereafter, the resulting powders were deposited manually into the wells of a slate plate (diameter 10 cm) with 207 positions to form the catalyst library (Fig. 1). Elemental analysis of selected samples showed residual carbon contents lower than 0.25 wt%, confirming the complete removal of organic material.

Catalyst library.
2.1.2 Bulk catalyst preparation
The conventional synthesis was performed following the same sol–gel process. For example, the Al1Mn6.7Co catalyst was prepared by adding 1872 μl of 4-hydroxy-4-methyl-2-pentanone into a continuously stirred beaker with 9129 μl ethanol. Afterwords 4615 μl Co(II)-propionate, 335 μl Mn(II)-propionate and 500 μl Al[C2H5CH(CH3)O]3 were added. The solution was stirred for 3 h followed by a drying period of 5 days at room temperature. Finally, the sample was calcined in air at 300 °C for 5 h.
The preparation of Co(II)-propionate has been described elsewhere [18]. Ni(II)-propionate and Mn(II)-propionate have been prepared similarly. Ni(OH)2 was refluxed (150 °C) with excess propionic acid. After getting a green solution the propionic acid was removed with a rotary evaporator and the remaining solid was dried at 130 °C. Mn(NO3)2
2.2 Catalytic measurements
2.2.1 High throughput screening
For the parallel detection of catalytic activity in combinatorial libraries, emissivity-corrected infrared thermography was used [5]. The set-up consisted of two infrared cameras the AEGAIS infrared camera from AIM equipped with a 256 × 256 PtSi-array detector and the PtSi 640 infrared camera of Thermosensorik GmbH in conjunction with an infrared reactor for catalysed gas-phase reactions. The infrared reactor consists of a steel housing with gas inlet and outlet connectors, in which the library is placed on top of the built-in electrical heater. The top of the reactor is sealed by a large sapphire plate, which allows us to monitor the infrared radiation of the plate by an infrared camera. Fig. 2 shows a picture of the infrared reactor.
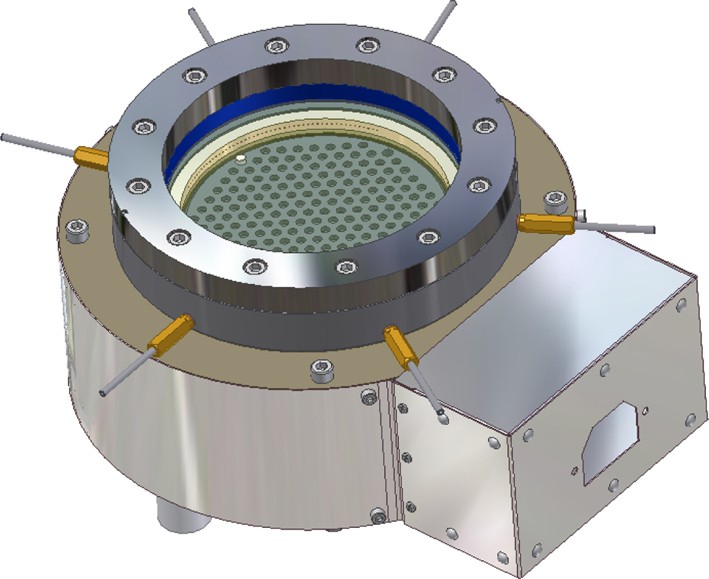
Infrared-thermography reactor with catalyst library under the transparent sapphire window.
The catalyst library was positioned in the infrared reactor and pre-treated in a flow of 50 ml min–1 air for 60 min at 200 °C. After cooling to 5 °C below the desired measuring temperature, a six-point calibration of the infrared camera was performed to correct the detector response and the emissivity differences of the materials in a library (pictures of the library were taken at temperatures of 5 °C below and above the desired reaction temperature by steps of 2 °C at an airflow of 49.5 ml min–1). Before starting the measurement an infrared image of the library was taken and subtracted as background. Such we could display temperature differences due to catalytic activities of materials in a library. The reactant flow consisted of 1 vol% CO in air at a total flow of 50 ml min–1. Infrared images of the libraries were recorded after different measurement periods. To compare the catalytic performances of the materials on one library, relative catalytic activities were calculated from the total heat response of each catalyst [19].
2.2.2 Conventional testing
The conventional experiments for the CO oxidation in air at 25 °C were performed in a fixed-bed reactor at atmospheric pressure. The reactor was a 6-mm i.d. glass tube inside of a furnace. One thermocouple was placed at the outside wall of the reactor to control the temperature of the furnace, another one inside the catalyst bed to measure the temperature inside the reactor. The feed gasses were adjusted by mass flow controllers. Gas analysis was performed by calibrated on-line gas sensors for CO, CO2 and O2. For calibration of the CO sensor, different gas mixtures of CO and CO2 in the range 0–1 vol% in air were measured. The corresponding output signals were related to the CO concentration (vol%) by a calibration curve. By adding CO2 to the gas standard, interferences of the CO sensor against CO2 could be corrected for. The calibration was checked by gas chromatography (60/80 Carboxen-1000, Supelco). 200 mg of catalyst (100–200 μm mesh) were placed on a quartz wool plug inside the reactor and pre-treated in a flow of 50 ml min–1 air for 60 min and afterwards in a flow of 50 ml min–1 N2 for 30 min, both at 200 °C. After cooling to 25 °C, the measurement was started to last for 60 min. The reactant flow consisted of 1 vol% CO in air at a total flow of 50 ml min–1. At the end of the catalytic test, the feed gas composition was measured to calculate the CO conversion as follows: XCO = ([CO]in – [CO]out/[CO]in) × 100%.
3 Results and discussion
3.1 Combinatorial approach
In this study, infrared thermography was used to search for new CO oxidation catalysts of high activity at low temperatures. There is a variety of theoretical strategies, such as factorial design, stochastic methods, evolutionary algorithms, neural networks, gradient methods, artificial learning, and others, which have been proposed for most efficient design of experiments in combination with high-throughput experiments [20–22]. When this project was started, these strategies were not yet available for practical applications. It was therefore decided to apply a more simple strategy based on selection and composition spreads.
In a combinatorial development the layout of the starting library is essentiel since it which sets the limits for all subsequent developments. While for discovery of completely new materials, a highly diverse nature of the starting library may be desirable, this also bears the high risk of failure. In a more conservative approach, a known system is selected and improved by high-throughput experiments. Here, we have chosen the latter strategy. It was decided early on to focus our search on noble-metal-free materials due to the economic aspect and the scientific challenge. From the literature certain CuOx-, NiOx-, CoOx- and MnOx-based materials are known to oxidize CO [11]. For true high-throughput experiments, material synthesis must be carried out by robot support. This required recipes for material syntheses compatible to our pipetting robot as well as to allow for highly diverse recipe variations. Such recipes based on a sol–gel process have been developed for the synthesis of NiOx-, CoOx-, and MnOx-based materials, while no such recipe could be developed in time for CuOx-based materials. Therefore, the starting library was based on the three former oxides in combination with a large number of doping elements supposed to improve catalytic performance. In detail the each base oxide was doped with 54 different elements and the remaining two base oxides in order to create a high chemical diversity (Fig. 3). This led to 56 binary mixed oxides of each base oxide prepared in two different doping concentrations. The material sols were prepared by a pipetting robot controlled by our library design software Plattenbau. The sols were dried and calcined and the powders were transferred manually to the wells of the library plates. In order to compare all libraries, several wells were filled with an industrial reference catalyst (Hopcalite). Initial goal was to identify those dopants that increase the CO oxidation activity of the single oxides. The catalytically most active materials were selected and their catalytic activity was optimised in subsequent libraries by binary composition spreads. The most active binary catalysts were then doped again and tested. The most active ternary catalysts were then optimised in a subsequent library by mapping out the ternary composition spread.

Fifty-seven different elements E of the periodic table used for the combinatorial experiments.
For the simultaneous evaluation of catalytic activity on materials, emissivity-corrected infrared thermography was chosen. With infrared thermography, heats of reaction are monitored under reaction conditions and provide excellent information on the relative catalytic activity of all materials on a catalyst library. It is one of the fastest methods for parallel activity detection. A disadvantage is the camera calibration time of about 2 h which is required for background correction and temperature recognition as well as for the recognition of false positives resulting from parallel or secondary reactions. In the case of CO oxidation, the latter is not a problem. Another source of false positives is the irreversible change of emissivity properties by adsorption/chemisorption phenomena on the catalyst surface. This is detected readily by switching off one of the reactant gases. If the observed heat of reaction does not disappear, a material change unrelated to catalytic activity has occurred [6]. The relative heat of reaction of all catalysts was determined by a software program, in which the heat spots on the infrared image associated with each material were integrated and normalized to the most active spot on the plate. Most active materials were thus identified by their location.
The results of such primary screening experiments were validated by preparing the materials of interest in bulk followed by testing under conventional gas-phase flow conditions. For reference control experiments with the industrial Hopcalite catalyst were carried out.
The general notation of mixed oxides prepared is DdCcBbA, where the capitals stand for chemical elements used in the synthesis and the subscripts for the amount of the corresponding element in mol%. For example, Ag3.3Mn34.1Co1.7Ni consists of mixed oxides of 3.3 mol% Ag, 34.1 mol% Mn, 1.7 mol% Co, and 60.9 mol% Ni. It was later recognized that the concentration of the Mn-precursor solution used was higher than initially anticipated. This error has been corrected in retrospect, leading to the non-integer nature of the material compositions and composition spreads.
3.2 Screening results of the starting libraries
The primary oxides NiOx and CoOx were doped with 56 different elements E in a quantity of 3 mol% and 10 mol% to create the binary mixed oxides E3Co, E10Co, E3Ni and E10Ni. MnOx was doped with 1.8 mol% and 6 mol% of each element E to get E1.8Mn and E6Mn. The higher doping levels were not investigated for noble metals and some alkaline and earth alkaline metals. These 306 different binary mixed oxides were prepared and positioned on two libraries. Fig. 4 displays the infrared images of the first library with E3Co, E10Co, E3Ni and E10Ni during CO oxidation at 50 °C and 100 °C. At 50 °C only the two mixed oxides Mn3Co and Mn10Co showed a remarkable catalytic activity. The relative catalytic activity of Mn3Co (100%) was higher than that of Mn10Co (66%). The performance of the other samples was negligible. This led to the selection of an Mn/Co composition spread on the next library. The temperature increase to 100 °C was accompanied by the appearance of more oxides with catalytic activity. Table 1 summarizes the 15 best materials. Four different binary mixtures of Mn, Co and Ni belong to the most active oxides. Therefore, mixed oxides of Mn, Co and Ni were also selected for the next library. The other starting library with samples of E1.8Mn and E6Mn mixed oxides showed no catalytic activity at 50 °C. These results indicate that catalysts of high Mn content lead to catalysts inactive at low temperatures, while mixing of Co with a smaller amount of Mn is beneficial for low temperature CO oxidation catalysis.
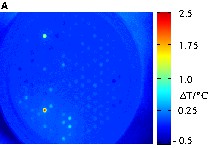
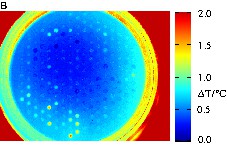
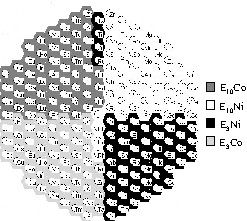
Screening of the catalyst library with E3Co, E10Co, E3Ni and E10Ni binary mixed oxides. (a) Emissivity-corrected infrared image at 50 °C after 60 min during CO oxidation. (b) Emissivity-corrected infrared image at 100 °C after 30 min during CO oxidation. (c) Positions of the mixed oxides in the slate plate.
Ranking of the 15 best samples of the catalyst library with E3Co, E10Co, E3Ni and E10Ni binary mixed oxides for CO oxidation at 100 °C after 30 min
Composition | Relative catalytic activity (%) | Composition | Rel. catalytic activity (%) |
Mn3Co | 100 | Mn10Co | 52 |
Si3Co | 80 | Rb3Co | 51 |
Na3Co | 78 | Re10Co | 48 |
Ti3Co | 63 | Ni10Co | 42 |
Ta3Co | 60 | Ni3Co | 40 |
Cs3Co | 53 | Ce3Co | 40 |
Zr3Co | 52 | Zn3Co | 37 |
Al3Co | 52 |
3.3 Combinatorial development of Mn–Co-based catalysts
MnxCo catalysts with Mn loading in the range of x = 1.7–16.1 mol% were prepared on the next library and tested in parallel. The results summarized in Table 2 show for Mn6.7Co the highest relative catalytic activity; therefore it was chosen as lead compound for further improvements.
Relative catalytic activity of MnxCo mixed oxides for CO oxidation at 50 °C after 15 min
Composition | Rel. catalytic activity (%) | Composition | Rel. catalytic activity (%) |
Mn1.7Co | 39 | Mn9.9Co | 96 |
Mn3.4Co | 80 | Mn11.5Co | 70 |
Mn5.1Co | 69 | Mn13.1Co | 61 |
Mn6.7Co | 100 | Mn14.6Co | 35 |
Mn8.3Co | 75 | Mn16.1Co | 43 |
Mn6.7Co-based ternary mixed oxides were synthesised by doping with 1 and 2.9 mol% of the remaining 55 different elements and their activity was tested by infrared thermography. The relative activity of the 15 best materials for CO oxidation at 50 °C is summarized in Table 3.
Ranking of the 15 best samples of the catalyst library with E1Mn6.7Co and E2.9Mn6.7Co ternary mixed oxides for CO oxidation at 50 °C after 120 min
Composition | Rel. catalytic activity (%) | Composition | Rel. catalytic activity (%) |
Mn6.7Co | 100 | Mg1Mn6.7Co | 56 |
Al2.9Mn6.7Co | 91 | Si2.9Mn6.7Co | 53 |
Mn6.7Co | 85 | Cd1Mn6.7Co | 52 |
Ga1Mn6.7Co | 83 | Ag1Mn6.7Co | 52 |
Ca1Mn6.7Co | 79 | Fe1Mn6.7Co | 52 |
Ge1Mn6.7Co | 69 | Na1Mn6.7Co | 52 |
Cs1Mn6.7Co | 67 | Al1Mn6.7Co | 47 |
Na2.9Mn6.7Co | 57 |
While the most active catalyst is still the binary mixed oxide, the doping elements appear to have no activating or deactivating effects. The experimental reliability is demonstrated by the activity of the two identical Mn6.7Co samples. One possibility for the slightly different relative catalytic activities of both Mn6.7Co samples could be different filling heights of the catalysts in the wells of the slate plate. To better evaluate the effect of the doping elements, the most active were selected and synthesized as ternary composition spreads ExMnyCo with E = Al, Na, Ga, Ge, Ca and Ag on the next library. Each composition spread consisted of 28 samples, with x = 0.4–2.7 mol% and y = 6.4–10.2 mol%.
During the following screening by infrared thermography, activity differentiation became difficult, because the high catalytic activity of all samples was similar and resulted in an activity gradient across the library. Apparently, due to high conversion at the reactor inlet side. The CO-decreased and caused a drop in reaction rate towards the outlet side of the reactor, which made reliable activity assignments on the library impossible. The problem could be overcome by decreasing the amount of oxygen in the feed gas to 1 vol% and increasing the amount of CO to 2 vol%. As an indicator for a uniform gas distribution in the infrared thermography reactor, several samples of the Hopcalite reference catalyst were placed at the gas inlet and outlet sides. Fig. 5a shows the corresponding infrared image during CO oxidation at 44 °C (inlet left, outlet right). The 7 hopcalite samples on the library showed, with a variation between 87 and 100%, the highest relative catalytic activity as well as an indication of the experimental error (most likely due to fill-height variations). As a reference, Mn6.7Co was placed twice on the library, giving at the lower left of the reactor a relative catalytic activity of 77% and at the upper right no activity. The most active ternary composition spreads were AlxMnyCo and AgxMnyCo. However, the ternary composition spreads with a lower activity (GaxMnyCo, GexMnyCo, CaxMnyCo) were those close to the gas outlet of the reactor. Fig. 5b shows the graph of the samples of the ternary composition spread of AlxMnyCo relating to the relative catalytic activity. It can be seen that the most active mixtures with a comparable activity to Hopcalite are Al0.8Mn8.3Co (86%), Al1.2Mn8.3Co (86%) and Al0.8Mn7.7Co (84%). Thus, it can be concluded that a low amount of Al (approx. 0.5–1.5 mol%) improves the catalytic activity of Co based oxides with a low content of Mn (approx. 7.0–9.0 mol%). Higher Mn and Al loadings result in a lower relative catalytic activity. However, comparing these results to AlxMn6.7Co (x = 1 and 2.9 mol%) in the other library, Al2.9Mn6.7Co showed a higher relative catalytic activity than Al1Mn6.7Co (Table 3), which is not consistent with the results of the ternary composition spread, indicating that we may have reached the confidence level of our primary screening technology.
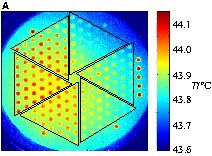
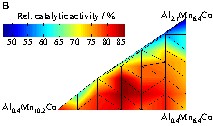
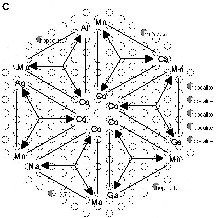
Screening of the catalyst library with ternary composition spreads of ExMnyCo with E = Al, Na, Ga, Ge, Ca, Ag and x = 0.4–2.7 mol%, y = 6.4–10.2 mol%. (a) Emissivity-corrected infrared-image at 44 °C during CO oxidation. (b) Graph of the relative catalytic activity of AlxMnyCo relating to the composition of the mixed oxides. (c) Positions of the mixed oxides in the slate plate.
On the starting libraries, the mixed oxides Si3Co, Ti3Co, Zr3Co and Al3Co also showed a high relative catalytic activity at 100 °C (Table 1). In addition, Al1Mn6.7Co, Al2.9Mn6.7Co and Si2.9Mn6.7Co exhibited a good catalytic performance (Table 3). Therefore, different loadings of E = Al, Zr, Ti, Si for ExMn6.7Co in the range of x = 1.0–9.7 mol% were investigated on another library and compared to the activity of Hopcalite. In Fig. 6, the relative catalytic activity is plotted against dopant concentrations. Such plots are especially valuable, since experimental errors are spotted easily. Increasing contents of Al, Ti, Zr and Si lead to a continuous decrease in relative catalytic activity in all cases. The best results were obtained by doping Mn6.7Co with Al. The most active sample was still Al1Mn6.7Co (100%) with a catalytic activity comparable to that of Hopcalite (98% and 89%).
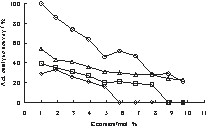
Relative catalytic activity at 50 °C of ExMn6.7Co mixed oxides with E = Al, Zr, Si, Ti and x = 1.0, 1.9, 2.9, 3.9, 4.9, 5.8, 6.8, 7.8, 8.8, 9.7 mol%. (o) AlxMn6.7Co, (Δ) ZrxMn6.7Co, (□) SixMn6.7Co, (◊) TixMn6.7Co.
Regarding the improvement of Mn-Co based oxides with Al doping, a low content of Mn (approx. < 9.0 mol%) and Al (approx. < 1.5 mol%) provide the best materials for low temperature CO oxidation. The low activity of 47% of Al1Mn6.7Co compared to 91% of Al2.9Mn6.7Co in Table 3 may be due to an experimental error, since it does not correlate with the trend in Fig. 6.
Al1Mn6.7Co and AlxMn8.3Co (x = 0.8 and 1.2 mol%) mixed oxides, with a catalytic activity comparable to Hopcalite, were discovered. At this point, it was decided to stop the combinatorial screening for Mn–Co based mixed oxides for low temperature CO oxidation and Al1Mn6.7Co was selected for further investigations at conventional testing conditions (see below).
3.4 Combinatorial development of Mn–Co–Ni mixtures
During screening of the first library at 100 °C (Fig. 4) for CO oxidation, good catalytic activity was observed for the binary mixtures Ni3Co, Ni10Co, and Mn10Co. Therefore, the mixed oxides of Ni, Mn and Co were chosen for a more detailed study. A ternary composition spread MnxCoyNi (1) with 66 samples in the range 0–100 mol% for each component was prepared and screened. Fig. 7 shows the infrared image of this library for CO oxidation at 50 °C and the positions of the samples on the plate. Clearly, the single oxides show the lowest activities and all three elements contribute to catalytic activity increases. High Mn contents (> 50 mol%) are unfavourable for the catalytic performance. This corresponds to the lack of activity of the E1.8Mn and E6Mn mixed oxides on the starting libraries (Fig. 7). Fig. 8a shows the graph of the 66 samples of the first ternary composition spread in relation to the relative catalytic activity. The most active mixed oxides were some binary oxides with no Co (Mn30.2Ni, Mn42.5Ni, Mn16.1Ni) and ternary oxides with a Co content below 20 mol% (Mn30.2Co8.7Ni, Mn16.1Co9.3Ni, Mn16.1Co18.6Ni). A second ternary composition spread was prepared, which amplified the most active area of the first composition spread. MnxCoyNi (2) with 66 samples in the range of x = 16.1–42.5 mol% and y = 0.0–18.6 mol% have been prepared and screened by infrared thermography for CO oxidation. Fig. 8b shows the graph of the 66 samples of MnxCoyNi (2) in relation to the relative catalytic activity for CO oxidation at 50 °C. The most active mixed oxides are Mn35.3Co1.7Ni (65%) and Mn37.8Ni (62%).
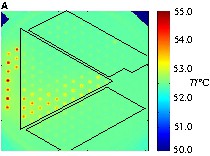

Screening of the catalyst library with M6Mn, M1.8Mn and MnxCo (x = 1.7–16.1 mol%) binary mixed oxides and the ternary composition spread MnxCoyNi (1) with x, y = 0–100 mol%. (a) Emissivity-corrected infrared image at 50 °C during CO oxidation after 20 min. (b) Positions of the mixed oxides in the slate plate.
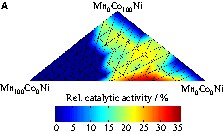
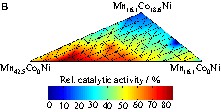
(a) Graph of the relative catalytic activity of Al xHn yCo relating to the composition of the several mixed oxides of MnxCoyNi (1) with x, y = 0–100 mol% during CO oxidation at 50 °C. (b) Graph of the relative catalytic activity relating to the composition of the several mixed oxides of MnxCoyNi (2) with x = 16.1–42.5 mol% and y = 0.0–18.6 mol% during CO oxidation at 50 °C.
Both catalysts, Mn35.3Co1.7Ni and Mn37.8Ni, were selected and doped with 54 different elements E to obtain ternary (E1.7Mn37.1Ni) and quaternary (E1.7Mn34.7Co1.7Ni) mixed oxides. In the case of Ag-doping, the compositions of the samples were Ag3.3Mn34.1Co1.7Ni and Ag3.3Mn36.5Ni. The library was screened for CO oxidation at 50 °C and the relative catalytic activity was compared to Hopcalite. Table 4 shows that the activity could be improved by doping the mixed oxides with Ag. The most active catalyst was Ag3.3Mn34.1Co1.7Ni (91%), with an activity comparable to that of Hopcalite (98% and 89%). The different relative catalytic activities of both Hopcalite samples are attributed to different filling heights in the wells of the slate plate. Ag3.3Mn34.1Co1.7Ni was selected as another promising candidate for conventional validation experiments (see below).
Ranking of the 10 best samples of E1.7Mn34.7Co1.7Ni and E1.7Mn37.1Ni mixed oxides for CO oxidation at 50 °C after 15 min
Composition | Rel. catalytic activity (%) | Composition | Rel. catalytic activity (%) |
Hopcalite | 98 | Cd1.7Mn34.7Co1.7Ni | 55 |
Ag3.3Mn34.1Co1.7Ni | 91 | Cs1.7Mn34.7Co1.7Ni | 54 |
Hopcalite | 89 | Ce1.7Mn34.7Co1.7Ni | 52 |
Cu1.7Mn34.7Co1.7Ni | 58 | Ge1.7Mn34.7Co1.7Ni | 51 |
Ag3.3Mn36.5Ni | 57 | B1.7Mn34.7Co1.7Ni | 50 |
3.5 Conventional validation
To validate the reliability of the combinatorial evaluation of catalytic materials, the combinatorial catalyst development was reproduced by selected conventional experiments. The base oxides of Co, Ni and Mn, Mn35.3Co1.7Ni as the best oxide of the second generation on the way to the final catalyst Ag3.3Mn34.1Co1.7Ni have been prepared in bulk by the sol–gel method as well as the two best catalysts discovered, Ag3.3Mn34.1Co1.7Ni and Al1Mn6.7Co. The activity of these catalysts was investigated for CO oxidation at 25 °C in the fixed-bed gas phase flow reactor. The results after 60 min of steady-state catalysis, shown in Fig. 9, nicely follow the trend expected from the high-throughput experiments. The activity of the Mn- and Co oxides prepared by the sol–gel method confirms that the recipe used provides active catalysts. The NiOx prepared by the same method shows an initial activity, which disappears already after a few minutes of reaction (not shown). The second-generation catalyst Mn35.3Co1.7Ni shows a significant increase to 57% conversion, but this is still improved considerably by Ag-doping. Ag3.3Mn34.1Co1.7Ni is the most active catalyst in this line of development, with a conversion of over 90% after 60 min steady-state catalysis.

Conventional verification of the combinatorial development of Ag3.3Mn34.1Co1.7Ni. 200 mg catalyst (100–200 μm mesh), 1 vol% CO in air, flow 50 ml min–1.
Mixing of Ni, Co and Mn had a beneficial effect on the catalytic activity. These results show that the evaluation of multi-component oxides on the basis of infrared thermography experiments was leading to a highly active catalyst, whose catalytic performance could be confirmed under realistic testing conditions.
In Fig. 10, the CO conversion of the two best catalysts, Al1Mn6.7Co and Ag3.3Mn34.1Co1.7Ni, is compared to that of the Hopcalite reference. Under our test conditions, both catalysts show a higher conversion than the Hopcalite at 25 °C. The most active catalyst found therefore is Al1Mn6.7Co (XCO > 90%), which makes it a promising candidate for room temperature CO oxidation.

CO conversion over Al1Mn6.7Co, Ag3.3Mn34.1Co1.7Ni and Hopcalite at 25 °C after 60 min. 200 mg catalyst (100–200-μm mesh), 1 vol% CO in air, flow 50 ml min–1.
4 Conclusion
Variation and selection, the basic principles of evolution, have been applied to catalyst development. The combinatorial strategy, doping, selection and composition spreads, has led rapidly to the discovery of new CO oxidation catalysts. Five catalyst libraries with more than 800 different multi-component oxides have been prepared by a sol–gel process with high-throughput techniques and their catalytic properties for low temperature CO oxidation activity were studied by emissivity-corrected infrared thermography. A variety of promising new ternary mixed oxides, free of noble metal, have been discovered, which oxidize CO at low temperatures. The two best materials are significantly more active under the laboratory test conditions than the industrial reference Hopcalite. It has been shown that the development system of doping, selection and composition spread is a very efficient procedure for combinatorial optimisation of catalysts. Within three generations of catalyst libraries, the poor activity of Co oxide could be dramatically improved to provide highly active new catalysts for low temperature CO oxidation. The composition of this catalyst has been preoptimized by the combinatorial approach; however, the reliability limit of the high-throughput technology has apparently been reached. For practical application a composition fine-tuning under conventional conditions and a variation of the recipe for optimisation of microstructure, pore size and porosity are advisable and may most likely result in further improvements. Our strategy of doping, selection, composition spread, selection, doping, selection, composition spread and selection can be recommended for the rapid improvement of catalytic properties.
Acknowledgements
This work was supported by the BMBF (FKZ 03C0311). The authors are grateful to K. Ammann from Dräger Safety AG & Co KGaA for a sample of the catalyst Hopcalite.