1 Introduction
New surfactants made of the association of anionic and cationic moieties so-called catanionic surfactants are currently developed [1,2]. They spontaneously form vesicles and can be used as a drug delivery vector [3]. Membrane fusion and endocytosis are the main possible mechanisms involved in the internalisation process. Fusion induces a direct release of the medicine into the cell core [4]. Endocytosis involves internalisation of the drug via small vesicles called endosomes [5]. Improvement of a drug vector needs a good understanding of the mechanism involved during the interaction of the vector with the cell membrane. Giant unilamellar vesicles (GUVs) are often used as cell models due to their ability to fusion [6], fission [7], or change their shape [8–10], for example when they are in interaction with external ionic species [8] or peptides [11]. Interaction with surfactants has been especially studied because they are known to solubilise lipidic membranes [12]. We showed that the initial step of the solubilisation of a lipidic membrane by a non-ionic surfactant as octyl glucoside involves the formation of transient pores [13]. The group of Brochart studied the interaction between lipidic membranes and Tween 20 below its cmc and showed that the surfactant stabilizes pores because of its preferential adsorption onto the edges of these pores in order to decrease the line tension [14]. Usually these studies involve low concentrations of surfactants, namely below the cmc. Electrostatic interaction of GUV with vesicles through fusion processes induced strong deformations of GUV and a decrease of 2 orders of magnitude of the membrane tension measured by analysis of the fluctuation spectrum [15]. Indeed we compare here the effect of a catanionic surfactant, denoted Tricat (Fig. 1), on magnetic GUV (GUV encapsulating colloidal maghemite nanoparticles) below and above the cac of the surfactant.
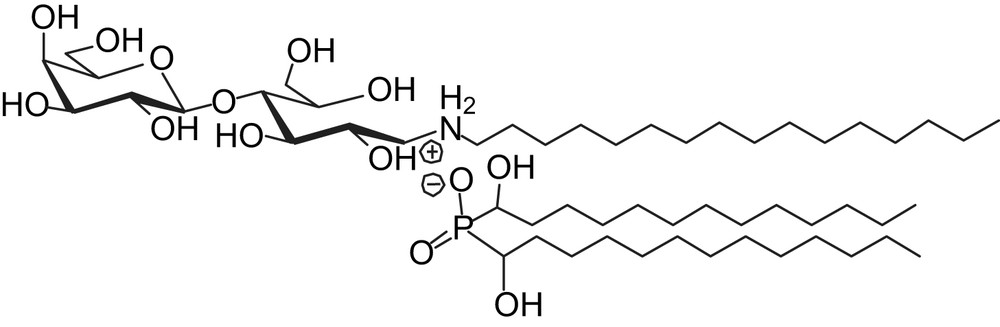
The so-called TriCat surfactant.
We also use magnetic GUV in order to test the interaction between the catanionic vesicles and lipidic membranes and thus evaluate the possibility to use catanionic vesicles as drugs carriers.
2 Experimental section
Citrate coated anionic maghemite nanoparticles, with a mean diameter of 7 nm, have been synthesized according to a previously described procedure [16]. They are stabilized by the so-called electrostatic repulsion between grains. The negative surface charges are due to ionized citrate species adsorbed onto particles and a residual ionic strength is due to unadsorbed citrate species, in equilibrium with the adsorbed ones. The ionic strength is deduced from conductivity measurements; the volume fraction of particles is deduced from the iron concentration, which is determined by atomic absorption spectroscopy.
The preparation of magnetoliposomes by spontaneous swelling has already been described elsewhere [17]. The preparation of liposomes by spontaneous swelling usually leads to a large variety of liposomes in terms of lamellarity, encapsulation rate and size. Nevertheless, we found that the quality of the obtained GUVs could be significatively improved by the control of the ionic strength of the initial magnetic fluid. Fig. 2a is then typical. But some of the spherical colorless vesicles in Fig. 2a are multilamellar vesicles and are not GUVs. Encapsulation of the magnetic fluid inside liposomes was performed using a spontaneous swelling procedure. A small mass of perfectly dry DOPC powder (around 1 mg) was placed in a glass Petri dish. Ten microliters of the magnetic fluid was added ([Fe] = 1.8 mol L−1; conductivity around 1 mS) and the mixture was spread and sheared with a glove finger to obtain a fat and oily orange film. Immediately following the shearing, 1.5 mL of distilled water was poured onto the fatty film to start the spontaneous swelling of the liposomes and the samples were placed in a water bath at 45 °C for 20 min. Due to the dilution of the magnetic fluid, the citrate concentration in the liposome solution is 1.9 × 10−4 mol L−1.
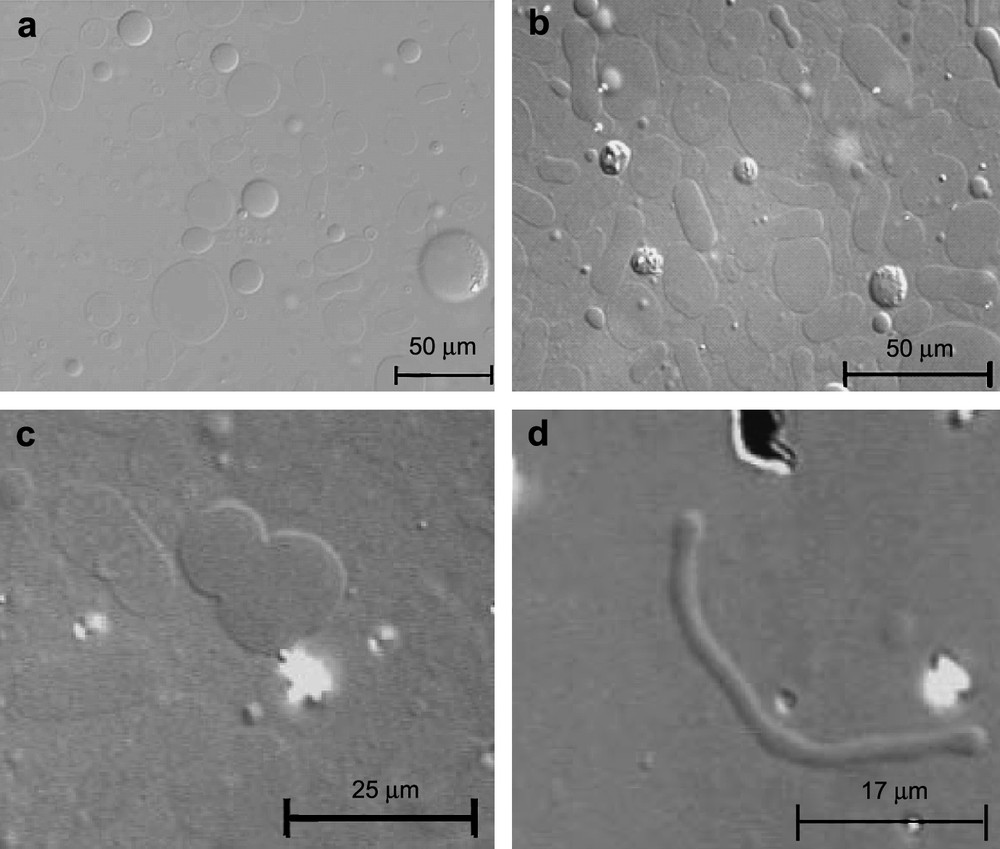
Optical microscopy images (Nomarsky contrast DIC) of (a) magnetic GUV; (b) magnetic GUV, 5 min after the addition of TriCat vesicles; (c) pears; (d) tubes.
TriCat surfactant (1-N-hexadecylammonium-1-deoxylactitol-bis(α-hydroxydodecyl) phosphinate), which spontaneously forms small vesicles in solution (100–500 nm), was synthesized via an acid–base reaction in an aqueous solution of trisodium citrate ([citrate] = 1.9 × 10−4 mol L−1) by addition of a phosphinic acid to an aqueous solution of a N-hexadecylamino-1-deoxylactitol [4].
Aqueous solutions of GUV ([DOPC] ∼ 3 × 10−3 mol L−1, 30 μL) and catanionic vesicles ([TriCat] = 1 × 10−3 mol L−1, 30 μL) were synthesized separately with the same trisodium citrate concentration, and then mixed (t = 0) before observation.
Because the shape modifications of the GUV appear very quickly after the mixing, it can be very difficult to observe them. Thus we used an observation cell where catanionic vesicles can be added to the GUV through a cut-off membrane of 800 nm, i.e. below the size of vesicles. A slow diffusion of TriCat vesicles is ensured and TriCat–GUV interactions can be observed as soon as GUV and catanionic vesicles are mixed (t = 0). Otherwise, this special observation cell was not used to improve the contrast of images.
Samples were imaged by transmitted light microscopy using an inverted microscope (Zeiss 40×, NA 0.65).
3 Results
Vesicular systems are very sensitive to their physicochemical environment. Catanionic vesicles are stable at pH 7 and are usually studied in de-ionized media [1]. Magnetic GUV are also stable at pH 7 but in a rather high ionic strength due to the encapsulated colloidal dispersion of citrate coated nanoparticles. Magnetic GUV and catanionic vesicles can thus be mixed at pH 7 but ionic strength needs to be the same for both systems otherwise the mixing can lead to an osmotic stress. The ionic strength is thus imposed by the magnetic GUV because encapsulated citrate coated nanoparticles have to be maintained in equilibrium with citrate ions in solution ([citrate] = 1.9 × 10−4 mol L−1). Hopefully, dynamic light scattering (DLS) showed that the catanionic vesicles were stable in an aqueous solution of trisodium citrate until [citrate] = 1 × 10−2 mol L−1: the vesicles size distribution remained unchanged compared to vesicles suspended in a de-ionized solution, even 3 days after preparation.
3.1 Shape modifications in zero field
Fig. 2b illustrates the shape modification the most often observed when magnetic GUV, initially spherical (Fig. 2a), are in the presence of TriCat vesicles. According to the [TriCat]/[DOPC] ratio, pears (Fig. 2c) and tubes (Fig. 2d) can also be found.
These deformations appear as soon as TriCat vesicles are added (in the first few minutes) as illustrated in Fig. 3.
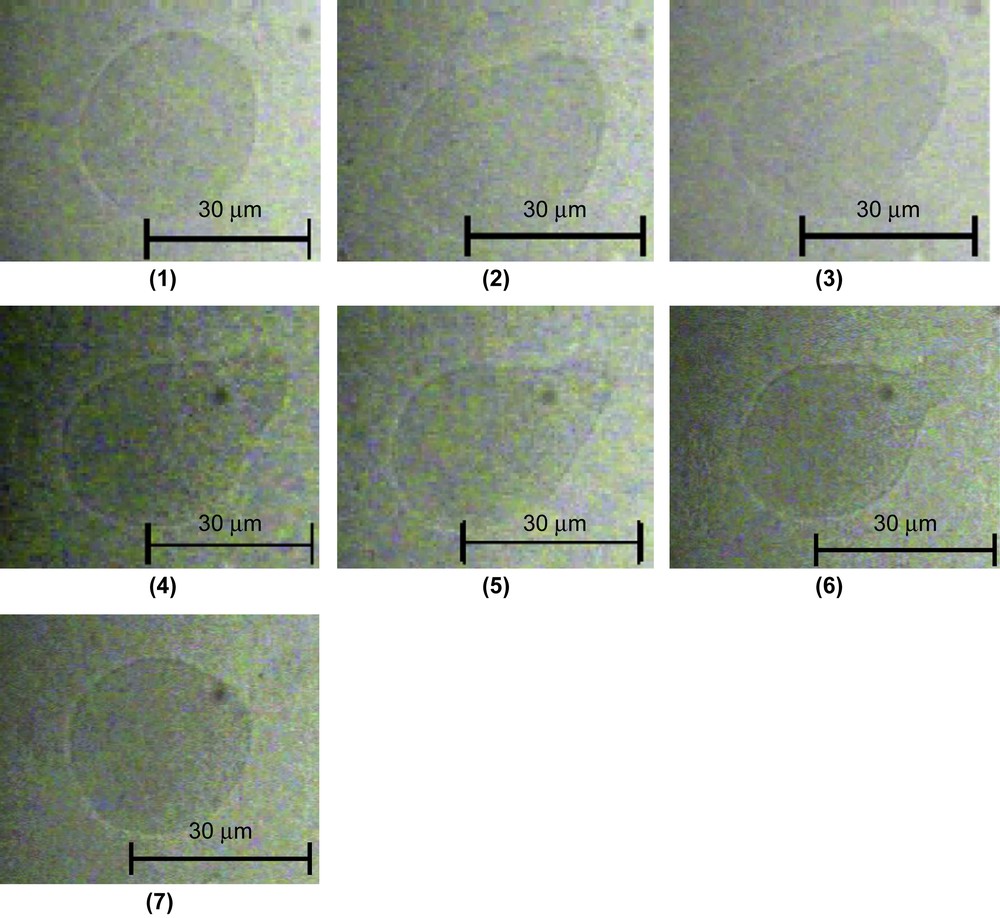
Shape change of a GUV induced by TriCat observed by transmitted light microscopy (Nomarsky contrast DIC) from a sphere to a pear shape before fission. The time after GUV and TriCat vesicles are mixed is (1) 0 s, (2) 2 min 20 s, (3) 2 min 21 s, (4) 2 min 42 s, (5) 2 min 47 s, (6) 2 min 57 s and (7) 3 min 5 s.
Pearling of the tubes has also been observed: small vesicles, all of them having the same diameter, appear first at the extremity of the tube, then to its centre in a very few minutes as illustrated by Fig. 4.

Shape change of a GUV induced by TriCat observed by transmitted light microscopy (Nomarsky contrast DIC) from a tube to a “pearl on a string” shape. The time after shape change is (1) 2 s, (2) 30 s, (3) 42 s, (4) 60 s, (5) 74 s and (6) 110 s.
The important point is that these topological modifications were not observed when TriCat was added in its monomeric state. GUV have been imaged after addition of the monomeric catanionic surfactant, for a concentration of TriCat in an aqueous trisodium citrate solution [TriCat] = 3 × 10−5 mol L−1, i.e. lower than the critical aggregation concentration of TriCat which is 5 × 10−5 mol L−1 (Fig. 5a). The magnetic GUVs remain spherical and the images are very similar to the ones obtained without TriCat.
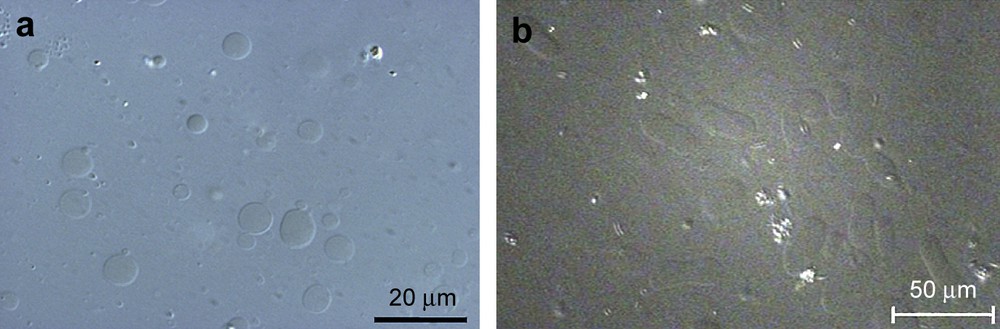
Optical microscopy images of (a) GUV with monomeric TriCat surfactant, [TriCat] = 3 × 10−5 mol L−1 and (b) GUV with TriCat vesicles, [TriCat] = 3 × 10−5 mol L−1 (Nomarsky contrast DIC).
Please note that for the same TriCat concentration, i.e. 3 × 10−5 mol L−1, obtained by diluting catanionic vesicles, without destroying them, important shape modifications are observed (Fig. 5b). For this experiment, the catanionic vesicles were synthesized above the CAC ([TriCat] = 1 × 10−3 mol L−1) and then diluted to obtain a surfactant concentration of 3 × 10−5 mol L−1; it has been checked by DLS that the TriCat vesicles were not destroyed during the time of the experiment and kept a size distribution similar to the one before dilution.
3.2 Effect of a homogeneous magnetic field
When a magnetic field is applied, the deformation of spherical magnetoliposomes into prolate ellipsoids is observed [18]. The prolate ellipsoid obtained is defined by the eccentricity, , where a is the semiaxis of the liposome parallel to the magnetic field, and b the value of the two other semiaxis orientated perpendicular to the magnetic field.
The basics of the model describing the deformation of isolated magnetic liposomes have been established previously in the case of a weak magnetic field [19], and were later extended to the case of ellipsoids of any elongation [20]. The eccentricity of the liposomes is linked to the applied magnetic field through the following expression
(1) |
(2) |
(3) |
(4) |
Theoretical curves that can be compared to experimental deformations are plotted in Fig. 6.
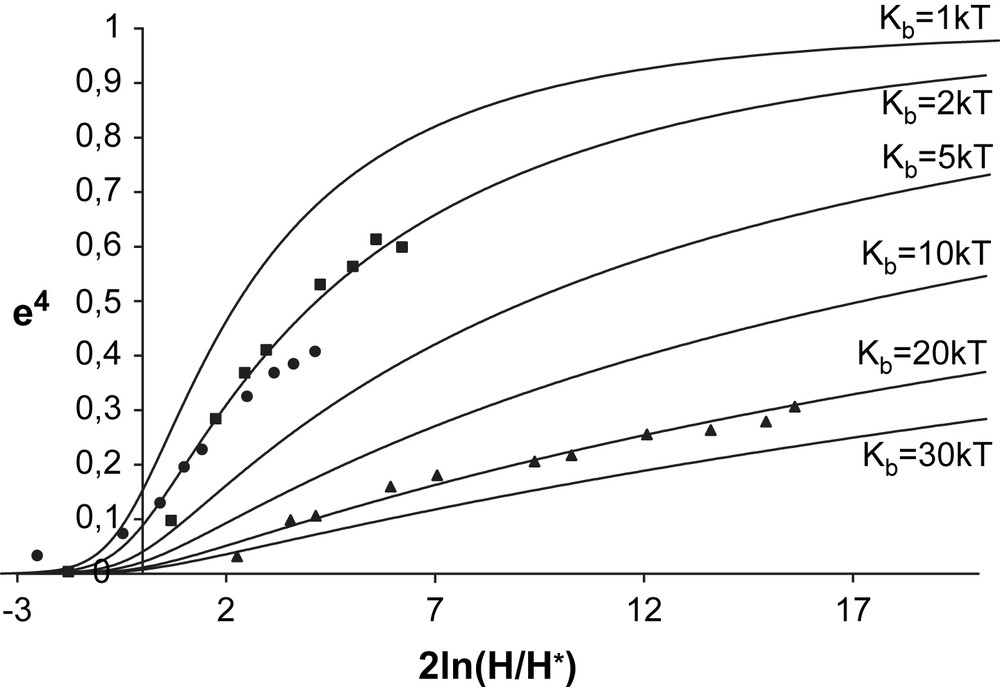
e4 versus the natural logarithm of the square of the reduced magnetic field, 2 ln(H/H∗). The symbols denote the experimental values of e4. The deformations of GUV without TriCat (triangles) were fitted by a theoretical curve calculated using Eq. (1) with Kb = 20kT, the deformations of GUVs in the presence of TriCat vesicles (squares) and monomeric TriCat (spheres) were fitted by a theoretical curve calculated using Kb = 2kT.
In the case where TriCat is added as a monomeric entity, we have seen that magnetic GUVs remain spherical. Nevertheless, when a magnetic field is applied, very important deformations are observed, corresponding to a bending modulus lowered down to 2kT compared to 20kT for GUV without TriCat.
The same kind of results is obtained for the few magnetic GUV staying spherical when TriCat vesicles are added: when the magnetic field is applied, they elongate to an ellipsoidal shape and come back to a spherical shape when the magnetic field is cut. But in this case, some liposomes present additional deformations, as illustrated by Fig. 7: they elongate in low field, but membrane instabilities can be perceived. Over a magnetic field of 200 Oe, a buckling of the membrane of the liposome is clearly observed. When the field is cut off, buckling disappears but the shape of the liposomes has definitively changed.
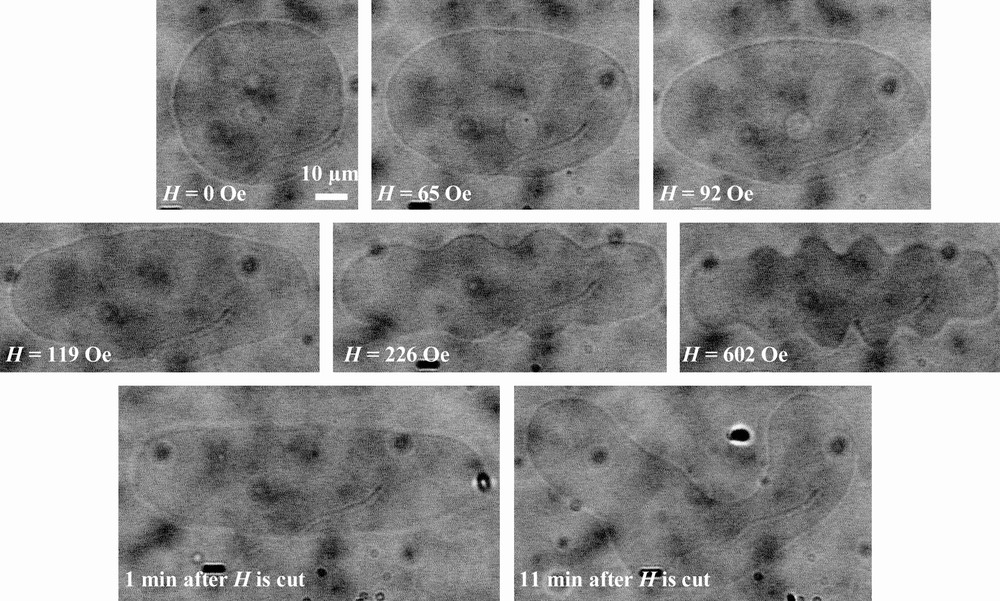
Deformation of a magnetic GUV in the presence of TriCat when a magnetic field is applied and after it is cut.
4 Discussion and conclusion
These results clearly show that TriCat strongly interacts with liposomes and that this interaction is related to the state of aggregation of this catanionic surfactant. In its monomeric state, it inserts into the membrane, inducing the decrease of the bending modulus. The liposome topology is not modified, indicating that its repartition inside the bilayer is symmetric.
The situation is clearly different when TriCat is under the form of catanionic vesicles. In this case the topology of liposomes is completely modified leading to elongated structures and tubes. In these modified GUVs, the membrane is close to instability: the tubes pearl in the absence of magnetic field, the membrane buckles when a magnetic field is applied.
Two models are generally used to describe the topological modification of liposomes, the area difference elasticity model (ADE) [21,22,23] which correspond to the insertion of the monomeric surfactant, and the phase separation model [24] corresponding to vesicles insertion.
The ADE model considers that each monolayer of the membrane has a fixed number of molecules and that the volume of the liposome is constant. At the equilibrium, the mean area A of the liposome is A = (Aext + Ain)/2, where Aext and Ain are the areas of the external and the internal monolayer of the membrane, respectively. Even if this mean area is a constant, monolayers are submitted to compression and expansion elastic energies. These energies can modify the value of ΔA = Aext − Ain which induces a deformation of liposomes. The ADE model determines the shape of liposomes with a minimisation of the elastic energy of the membrane with only two parameters: the reduce volume of the liposome v and the difference of area ΔA.
Considering the phase separation model, the first step is the fusion of vesicles with GUVs. The membrane becomes inhomogeneous if the surfactant is unable to diffuse and/or mix with the lipids. Observation of pears and pearling tubes is characteristic of this case of inhomogeneous membranes [25].
The fact that GUVs become tubes, discocytes or pears when TriCat vesicles are added, but remain spherical when monomeric TriCat is added, although elongation measurements show that the surfactant is in the membrane, indicates that the shape modifications are not due to an asymmetric repartition of the surfactant in the lipidic bilayer, and pleads for phase separation. The shape modifications are observed in the few seconds after the mixture; the membrane is close to instability, an applied magnetic field inducing pearling buckling at very low field. The excess of surfactant suddenly provided to the membrane has quite different consequences than the addition of monomer species, and it is reasonable to think that a demixing occurs, which seems irreversible during the time of observation (one day). Fluorescent TriCat has to be synthesized to fully elucidate the mechanism of interaction between TriCat vesicles and GUV. Nevertheless the results obtained here point out the important interaction between the TriCat vesicles and the lipidic membrane and are encouraging for the use of the latter as drug carriers.