1 Introduction
In 1996, J. Livage published in this journal the first review paper on bioapplications of sol-gel chemistry that gathered main achievements in the encapsulation of enzymes, immune molecules and whole cells [1]. At this time, bioencapsulation was an emerging field of application of sol-gel technology, initiated by the groups of G. Carturan and D. Avnir [2,3]. About 10 years after, biomolecule immobilization in sol-gel matrices has become a very popular method both for fundamental studies of enzyme reactivity and for the design of biosensors and biocatalysts [4–6]. The development of microorganism encapsulation has been significantly slower but recent reviews have enlightened the potentialities of this approach [7–9].
As in many other fields of application of sol-gel technology, silica has long been the only material studied as a host for bioencapsulation in general and whole cell entrapment in particular [10]. However, the successful immobilization and long-term viability of Escherichia coli bacteria in an aluminium oxyhydroxide gel (boehmite) was recently reported [11]. The encapsulation was performed using a novel colloidal route based on the preparation of nanoparticles from aqueous salts followed by gelation of the redispersed colloids induced by phosphate buffer (PB) addition. This methodology was very recently extended to iron oxyhydroxide (ferrihydrite) gels [12]. In parallel, it was possible to preserve the short-term viability of Picha anomala yeasts cells and Lactobacillus plantarum lactic acid bacteria coated with titanium oxide nanoparticles prepared using metal alkoxide micelles templated by self-assembly of ligands [13].
The question therefore arises whether the colloidal approach to cell encapsulation can be extended to all, or at least to a great variety of metal oxide gels. With this purpose, we report here the formation of zirconium-based colloidal gels following an aqueous route and their suitability for E. coli bacteria encapsulation. A comparison with other metal oxyhydroxide gels is provided, underlining that the surface reactivity of the starting nanoparticles is a key parameter for the success of the encapsulation procedure.
2 Experimental
2.1 Zirconium oxyhydroxide gel preparation and characterization
Zirconium oxyhydroxide (ZrO[OH]2) nanoparticles were prepared by the thermohydrolysis of a zirconium oxychloride salt in alkaline conditions. A 0.1 M sodium hydroxide aqueous solution was added dropwise into a 0.1 M aqueous solution of ZrOCl2 until pH = 8. The final zirconium concentration was 0.07 M. The resulting suspension was left at 95 °C over 1 week. At this stage, a gelatinous precipitate was recovered with a final solution pH of 5. The precipitate was centrifuged and washed several times with deionized water to get rid of unreacted salts. The electrophoretic mobility of the particles was measured at room temperature with an electrophoresis light scattering Zeta Plus instrument (Brookhaven Instruments Corp). The measurement was performed on particle suspensions at a 25 g/L concentration and a constant ionic strength I = 0.1.
Gels were obtained after resuspension of 2 g of the precipitate in 0.25 mL of deionized water and addition of 1 mL of 0.1 M PB as such or supplemented with 10% w/w glycerol (PB-gly). The resulting sols were rapidly homogenized with a vortex. A white opaque gel was obtained within a few seconds in the absence of glycerol. In contrast, the presence of glycerol led to a viscous suspension that does not gel over a few days and was not further studied.
Zirconium oxyhydroxide xerogels were obtained after freeze-drying. Their crystalline structure was studied by X-Ray Diffraction (XRD), using Panalytical X’pert Pro diffractometer equipped with a multichannel X’celerator detector, using Co Kα radiation (λ = 1.7889 Å) in the 2θ range of 10–80°. The porosity of xerogels was measured by nitrogen sorption experiments performed at 77 K on a Micromeritics 2010 sorptometer. Prior to analysis, samples were first degassed at 60 °C under a 3 μmHg pressure. The specific surface area SBET was determined by the Brunauer–Emmett–Teller (BET) method and the porous volume Vp calculated at the leveling off of the isotherms. Xerogels were also studied by scanning electron microscopy (SEM) using a JEOL model JSM-5510 at an accelerating voltage of 10 kV and by transmission electron microscopy (TEM) on a Philips CM12 electron microscope, operated at 120 kV.
2.2 Bacteria growth and encapsulation procedures
Stock cultures of E. coli cells were prepared from cultures stored at −80 °C in Luria-Bertani (LB) broth supplemented with glycerol. When needed, cultures of bacteria in LB broth were prepared overnight at 37 °C. An inoculum (0.1 mL) was added to a glucose (20 mM)-minimum phosphate broth (minimum medium MM) (50 mL) at pH 7 in a flask (250 mL) and grown at 37 °C under stirring (200 rpm). After 3 hours of incubation, corresponding to the mid-exponential phase of growth, the culture was harvested by centrifugation at 6000 rpm for 15 minutes at 9 °C. The pellet was washed twice with PB and diluted to reach a concentration of 109 cells/mL in PB (working cell suspension, WCS) or in PB-gly (WCS-gly).
Bacteria encapsulation in zirconium oxyhydroxide gels was performed following the above-described procedure using WCS and WCS-gly instead of PB and PB-gly, respectively. As already observed in the absence of cells, gels could only be obtained upon WCS addition whereas the presence of glycerol in the cell suspension prevents gel formation. For viability measurements, wet gels were aged for 1 hour at 20 °C in the mother solution in a closed flask. After this period, they were crushed, and vigorously stirred with PB (3 mL). A series of 10-fold dilutions of WCS or resuspended gels (0.1 mL) diluted in PB (0.9 mL) were surface-plated in triplicate on LB-agar. Plates were then incubated at 37 °C for 24 hours before performing the colony forming units (CFU) count.
The effect of zirconium oxyhydroxide particles on the growth of bacteria was studied in a MM solution. In a typical experiment, a bacteria inoculum (100 μL) was added to the culture medium MM (50 mL) in a flask (250 mL) and zirconium oxyhydroxide particles, at different concentrations (5, 2.5 and 0.5 g/L), were added to the cell suspension. Cells were incubated at 37 °C under stirring. The growth of bacteria was followed by turbidity measurements as the optical density (OD) of suspension aliquots recorded at λ = 600 nm is proportional to bacteria concentration.
3 Results and discussion
The alkalinization of a zirconium oxychloride aqueous solution is expected to induce the precipitation of a zirconium hydroxide phase that undergoes structural and chemical rearrangement during thermohydrolysis [14]. Here, a decrease in the pH of the solution suggests a partial conversion of hydroxyl groups into oxobridges, leading to a zirconium oxyhydroxide phase.
Upon PB addition, a stable gel is formed. In order to understand this process, the isoelectric point (pI) of obtained nanoparticles was determined by monitoring the variation of their zeta potential with pH (Fig. 1). The pI value was found to be 8.5 ± 0.5, indicating that the nanoparticles are positively charged at the pH of encapsulation. Therefore, it can be suggested that the anionic phosphate groups can act as screening species to reduce interparticle electrostatic repulsion and favor gel formation. It was noticed that this process was hindered by glycerol addition. In fact, glycerol was previously used for the synthesis and stabilization of zirconia nanoparticles due to its ability to adsorb on their surface through zirconium chelation [15]. It can therefore be suggested that such an adsorption prevents an efficient surface charge screening by phosphate ions and therefore hinders gel formation.

Variation of the zetapotential ζ of the zirconium oxyhydroxide nanoparticles with pH.
To evaluate the chemical composition of the obtained xerogels, elemental analyses were performed on Zr, Cl and P contents. They indicate a molar ratio Zr/Cl ≈ 0.15, to be compared with the initial Zr/Cl = 2 stoechiometry of the zirconium salt. This confirms its efficient conversion into an oxy/hydroxy phase and also the suitable washing of the particles.
The XRD pattern of the xerogels exhibits two broad peaks at 2θ = 37° and 59° (Fig. 2). This diffractogram is highly reminiscent of the XRD profile of an intermediate phase obtained during the thermal conversion of a nanostructured zirconium oxyhydroxide amorphous material into nanocrystalline tetragonal ZrO2 (t-ZrO2) [16]. In this context, it can be proposed that the two peaks at 37° and 59° correspond respectively to the (101) and (200)/(103) planes of a disordered, partially hydroxylated t-ZrO2 phase. In parallel, examination of the zirconium oxyhydroxide xerogel by SEM shows that the gel surface is granular, consisting of uneven aggregates of submicrometric particles (Fig. 3a). TEM observations suggest that these particles are porous and formed by a loose packing of much smaller plate-like nanoparticles, ca. 5 nm in size (Fig. 3b), although the rapid dehydration of these nanoparticles under the electron beam prevents a precise determination of their morphology. More information on the gel structure is obtained by nitrogen sorption measurements performed at 77 K. The isotherm, shown in Fig. 4, is typical of a type IV mesoporous material. The calculated specific surface area SBET and porous volume Vp are 400 m2/g and 0.41 cm3/g respectively. Noticeably, the shape of the hysteresis curve is of the H3 type, indicating that the xerogel consists of loose aggregates so that the material tends to swell with increasing nitrogen partial pressure [17]. Such a hysteresis shape is often observed for materials formed by the stacking of plate-like particles, in good agreement with the TEM observation. In such conditions, the BJH model cannot be accurately applied for pore size distribution calculation. An estimation of the average pore size Dp can nevertheless be obtained by simple calculation of the 4 Vp/SBET ratio, leading to Dp ≈ 40 Å.

X-ray diffraction pattern of zirconium oxyhydroxide xerogels.

(a) Scanning electron microscopy and (b) transmission electron microscopy images of the zirconium oxyhydroxide xerogels.
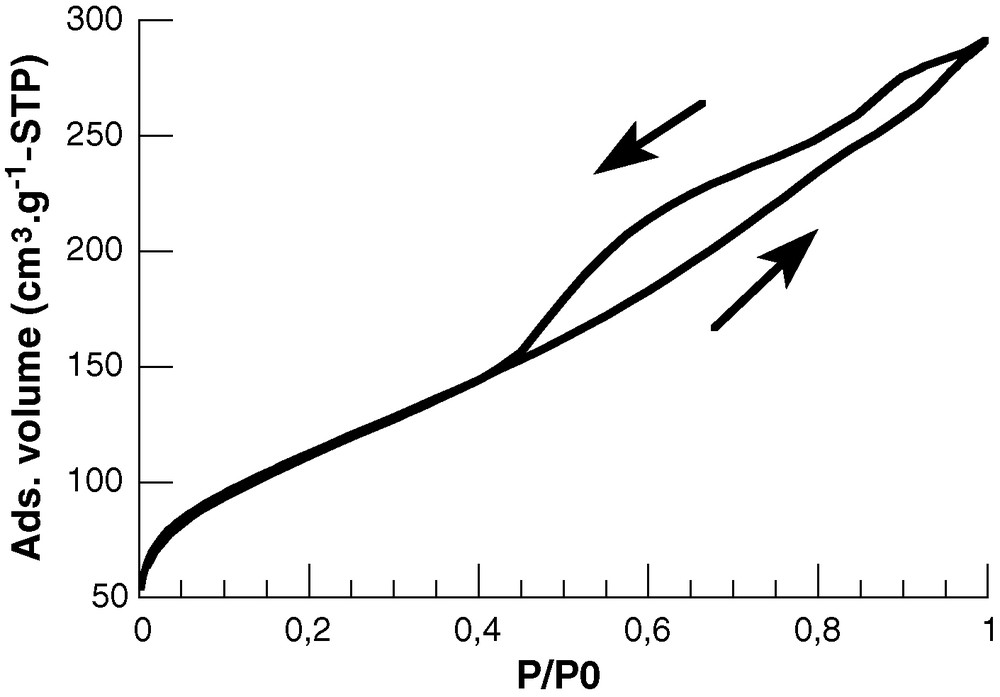
N2-sorption isotherm of the zirconium oxyhydroxide xerogels.
These gels were then evaluated for E. coli bacteria encapsulation. However, 1 day after encapsulation, the viability determined by the plate count technique indicates a survival rate of ca. 5%. As a comparison, the cell suspension showed a nearly 100% viability rate after the same delay. In order to try to understand this result, E. coli bacteria were grown in a minimum medium in the presence of increasing concentrations of zirconium oxyhydroxide nanoparticles (Fig. 5). Bacteria growth curves display a characteristic three-steps shape. The initial lag time increases with the concentration of nanoparticles, showing that bacteria are affected by the presence of the inorganic colloids in the medium. The log phase, during which bacteria growth is exponential, lasts 7 hours for all experiments, with no significant variation in the curve slope. However, the optical density measured at the plateau corresponding to the stationary phase, which occurs when the nutrients become limited, is decreased upon nanoparticle addition, suggesting that part of the bacteria have been killed at the contact of the zirconium oxyhydroxide suspension.

Influence of zirconium oxyhydroxide nanoparticles (Zr) concentration on E. coli growth in a minimum media (MM).
It is interesting to compare these data with previous reports on E. Coli encapsulation in boehmite and ferrihydrite colloidal gels [11,12]. In both cases, the viability after 24 hours was found to be ca. 30%. In Tables 1 and 2, we have gathered several parameters that may be relevant to understand the observed difference in encapsulated bacteria viability. Considering the features of the colloids, it is clear that neither the pI value [pI (FeOOH) < pI (ZrO(OH)2) < pI (AlOOH)] nor the particle shape (platelets for both Al- and Zr-oxyhydroxide) or dimensions (similar for Fe- and Zr-oxyhydroxide) provide an explanation for the observed difference in cell survival. In terms of xerogel porosity, it is to be noted that the three materials are mesoporous but the shape of the isotherm differs (H3 for zirconium oxyhydroxide compared to H2 for the two other xerogels) while the specific surface area, the porous volume and the average pore size are higher for the zirconium phase than for the other materials. Although this variation in the porous structure of the gel may have an impact on cell viability, it is difficult at this time to discuss this point further. Finally, in terms of nanoparticle suspension impact on E. coli growth, an increase in lag time is observed for all systems, the slope of the exponential curve is either decreased, increased or unmodified for high concentrations of boehmite, ferrihydrite and zirconium oxyhydroxide respectively. However, only in the case of the zirconium phase does a significant decrease in the optical density at the plateau is observed. Noticeably, this decrease is of about two orders of magnitude, i.e. about 1% of the initial bacteria population have successfully grown in the presence of the zirconium oxyhydroxide suspension. Interestingly, this value is in the same range as the observed viability rate of encapsulated cells (ca. 5%). Therefore, it can be suggested that such a low survival rate is related to the cytotoxicity of the nanoparticle suspension. This result is all the more surprising as Zr, zirconium hydroxide and zirconia are usually considered as non-toxic [18–20]. However, a straightforward comparison with the literature is rendered difficult due to the various conditions of media, particle size and concentration as well as the diversity of the chemical nature of the zirconium phase, all these parameters having a potential influence on cytotoxicity. Therefore, further studies are required to examine in more details the detrimental effect of our system on cell viability.
Properties of oxyhydroxide colloids and their impact on the different phases of E. coli growth.
Oxyhydroxyde | Colloid properties | Impact on bacteria growth | |||
pI | Size (nm)/Morphology | Lag time | Exponential phase slope | Stationary phase OD | |
Al(III)a | 9.5 | 100 nm/fibers | Increase | Decrease | Equal |
Fe(III)b | 7.2 | 5 nm/spheres | Increase | Increase | Increase |
Zr(IV)c | 8.5 | 5 nm/platelets | Increase | Equal | Decrease |
4 Conclusion
Our previous works have demonstrated that glycerol addition to AlOOH and FeOOH colloidal solutions leads to the formation of stable gels that could be used for E. coli bacteria encapsulation. The glycerol presence does not significantly impact on the short-term viability of encapsulated cells but favors their long-term survival [21,22]. In the case of boehmite and ferrihydrite, such a stabilizing effect allows the preservation of a reasonable survival rate of bacteria (ca. 15%) over one month, even though the viability after 24 hours is limited to 30%. In the present case, both the low short-term viability rate (ca. 5%), that may be attributed to colloid toxicity, and the impossibility to form gels in the presence of glycerol, due to its adsorption on nanoparticle surface, indicate that the present colloidal route is not adapted to the encapsulation of E. coli bacteria in zirconium-based gels. In many instances, the short-term cytotoxicity of nanoparticles is due to their interaction with cell membranes, and can therefore be controlled by a suitable surface modification [23]. In the present case, such a surface modification may also prevent glycerol adsorption. However, care should be taken that grafted moieties do not prevent gel formation via steric repulsion. In this context, we are currently studying the surface modification of our colloids with amino silanes [24].
On a more general level, the present work underlies some of the challenges involved in the application of an aqueous colloidal route to cell encapsulation in metal oxide gels. In fact,this route was designed to avoid the possible toxicity of metal alkoxides and of some metal ions, as well as to allow gel formation in biocompatible conditions (neutral pH, low ionic strength, moderate temperature, absence of organic solvents and/or by-products). However, this study reveals that the nanoparticles themselves can also present some cytotoxicity for which reliable data are difficult to find in the literature. The second limitation lies in the interaction between glycerol, a key additive for the long-term preservation of encapsulated bacteria, and the nanoparticles, that may prevent gel formation. In both cases, these challenges may be overcome via a suitable surface functionalization of the nanoparticles. This strategy will be the main guideline for our future developments in this area, with the hope of demonstrating that this colloidal route constitutes surely not a universal but at least a general method for bacteria encapsulation in inorganic gels.
5 Conflicts of interests
The authors declare no conflict of interests related to this work.