1 Introduction
Topology is a branch of mathematics dealing with the properties of geometrical forms that remain invariant under given transformations such as stretching or bending. In other words, the topological properties of a given object are not modified by continuous deformation of the object in a three-dimensional space as long as no link is broken. Since the metric properties of the object are of no importance as far as its topological properties are concerned, topology is completely different from Euclidian geometry.
The fascination exerted on molecular chemists by knots and catenanes stems originally from their topology. For instance, a simple catenane (i.e., a compound consisting of two interlocking rings) is unique in the sense that any projection of the molecule on a plane will have to contain two crossing points, regardless of the distortion that might be imposed on bonds and angles: its molecular graph, consisting of vertices (i.e., its atoms) and edges (i.e., the connections between the atoms or, in a chemical language, the chemical bonds) is nonplanar. Another way to say exactly the same thing is that the topological properties of the object are nontrivial. Paradoxically to the vast majority of molecular chemists, who are nowadays used to think in a three-dimensional fashion, molecules with nonplanar graphs are very uncommon. Even the most complex artificial or natural molecules have a planar graph: they are trivial from the viewpoint of a topologist! Their molecular graphs can be drawn in a plane (a sheet of paper) without crossing points between the lines connecting the atoms. This can easily be done by bending, stretching or contracting the bonds at will, which are perfectly allowed operations in topology. It may thus appear as highly surprising that C60 or double-stranded helices, such as those found in DNA fragments, are topologically planar, and thus topologically trivial molecules, but it is the case, in spite of the obvious three-dimensional nature of fullerenes and the helical arrangement of the two DNA strands.
Although the field of catenanes and rotaxanes is an old research field [1], it is only relatively recently that it became so active, mostly in relation to the novel properties that these compounds may exhibit (electron transfer, controlled motions, mechanical properties, etc.). In addition, catenanes represent attractive synthetic challenges in molecular chemistry. The creation of such complex functional molecules as well as related compounds of the rotaxane family demonstrates that synthetic chemistry is now powerful enough to tackle problems whose complexity is sometimes reminiscent of biology, although the elaboration of molecular ensembles displaying properties as complex as biological assemblies is still a long-term challenge. This field has interesting connections to that of controlled dynamic molecular systems, named “molecular machines”, which are molecular systems able to undergo large-amplitude motions under the action of an external signal. In the following review article we will mostly focus on the results obtained in Strasbourg, in the fields of molecular topology and molecular machines, although highly creative work has been carried out in several other research teams.
2 Copper(I)-templated synthesis of catenanes: the “entwining” approach and the “gathering and threading” strategy
The most efficient strategies for making such compounds are based on template effects. The first templated synthesis [2] relied on copper(I). The use of Cu(I) as template allows one to entangle two organic fragments around the metal centre before incorporating them in the desired catenane backbone. The strategies used are represented in Fig. 1. The first strategy A is very straightforward but will preferably lead to symmetrical catenanes consisting of two identical interlocking rings. The second approach B necessitates the preliminary synthesis of a coordinating ring but, on the other hand, catenanes consisting of two different rings can be made using this stepwise procedure. In Figs. 2 and 3 are shown the real molecules and the chemical reactions leading to the desired catenanes. It should be noticed that the 27% yield obtained originally for the first copper(I)-complexed catenane allowed one to prepare this molecule at the several hundred milligram scale. Meanwhile, the procedure has been gradually improved, culminating with a 92% yield for the double cyclisation reaction using the ring-closing metathesis methodology in collaboration with Grubbs and co-workers (Fig. 4) [3].

General transition metal-templated strategy; the black dot represents a transition metal (copper(I)); the arcs of a circle and the rings contain coordinating fragments. The success of the approach relies on the formation of an “entanglement” before the cyclisation reaction(s) is(are) carried out.

The first templated synthesis of a [2]catenane. The copper(I) complex obtained (“catenate”) is extremely stable due to the interlocking nature of the ligands.

Removal of the copper(I) centre and liberation of the free catenane ligand (“catenand”).

High-yield synthesis of a [2]catenane using the methodology proposed by Grubbs and his co-workers. The overall yield is close to quantitative [3].
Organic templates assembled via the formation of aromatic acceptor–donor complexes and/or hydrogen bonds have also been very successful. Hydrophobic interactions have also been exploited in a very successful manner. Nowadays, numerous template strategies are available which have led to the preparation of a myriad of catenanes and rotaxanes incorporating various organic or inorganic fragments and displaying a multitude of chemical or physical functions (Fig. 5) [4].

A beautiful representation of a trefoil knot from the Dutch artist, Maurits Cornelius Escher (1898–1972).
3 Molecular knots
Knots are fascinating non-trivial topological entities. They are not only present in art and history but in many scientific fields as well, ranging from mathematics to biology. By targeting this tantalizing structure, chemists have contributed to the promotion of their beauty. In mathematics, knots and links occupy a special position. They have been the object of active thinking for more than a century [5]. Any interested reader should have a look at a relatively recent and accessible small book entitled The Knot Book [6]. In Fig. 6 are represented the first 10 prime knots. These knots are single-knotted loops, in contrast to “links” (or catenanes, in chemistry), which are sets of knotted or unknotted loops, all interlocked together. The discovery that DNA forms catenanes and knots, some of them being of extreme complexity, initiated a new field of research that has been called “biochemical topology” [7]. Besides naturally occurring DNA catenanes and knots, a fascinating family of related molecules has been synthesized and described by Seeman and co-workers [8]. The elegant approach of this group utilizes synthetic single-stranded DNA fragments, which are combined and knotted by topoisomerases. Interestingly, DNA is not the only biological molecular system to have the privilege of forming catenanes and knots. Liang and Mislow examined X-ray structures of a large number of proteins and, to the surprise of many molecular chemists and biochemists, they found catenanes and even trefoil knots [9,10].

The 10 first prime knots. Even the simple 4-crossing knot 41 still remains to be made at the molecular level. 31, 51 and 71 are called “knots of the torus” (they can drawn on the surface of a torus without crossing points). They are topologically chiral, contrary to 41.
The trefoil knot occupies a special position among the knots: it is, of course, the simplest nontrivial knot and it has been used in art numerous times by many civilisations. A particularly aesthetically attractive representation of it is given in Fig. 5.
The success encountered in the synthesis of various catenanes following the strategy depicted in Fig. 1 led to a molecular trefoil knot synthesis by extending the former synthetic concept from one to two copper (I) ions [11]. As shown in Fig. 7, two bis-chelating molecular threads (A) can be interlaced on two transition metal centres, leading to a double helix (B). After cyclisation to (C) and demetalation, a knotted system (D) should be obtained. An important prerequisite for the success of this approach is the formation of a helical binuclear complex (B).

The strategy leading to a trefoil knot is represented on the bottom line. It involves two metal centres and two coordinating organic threads [11]. On the upper line, the synthesis principle of a [2]catenane is also given, to show the analogy between both strategies.
Using 1,10-phenanthroline derivatives, this approach turned out to be successful although the yield of the reaction was, at first, far from being satisfactory (3%) [11]. Gradually, it was improved by modifying key structural features of the precursors and the final double cyclisation reaction (Fig. 12) [12]. Once again, the ring-closing metathesis of olefins turned out to be spectacularly efficient, leading to a yield of 74% for the last double cyclisation step [13]. The sequence of reactions originally developed and affording the first molecular trefoil knot (1989) is indicated in Figs. 8, 9 and 11.

The use of the meta-phenylene connector between the two 1,10-phenanthroline nuclei of the same thread results in much better yields for the double stranded precursor and for the trefoil knot (30%) [12].

Formation of the double stranded helical precursor.

The dicopper-trefoil knot is obtained via a double cyclisation reaction from the double stranded helical precursor [11]. Since the isolation and the purification of this precursor seemed to be practically impossible due to the complexity of the mixture obtained by mixing the organic coordinating threads and the corresponding stoichiometric amounts of copper(I), the ring-forming reaction was performed on the crude mixture, containing only a relatively small proportion of the helical complex (∼15%). Once the topology is “frozen”, i.e., the ring has been closed, the compound is stable and can be isolated pure by chromatography. Several columns were required but, in spite of the very low preparative yield, amounts in the range of 20 to 70 mg could be obtained per reaction.

The complexed knot can be demetalated quantitatively to afford a chiral knotted ring, ready to undergo further coordination chemistry with a variety of cationic metal centres, as shown by subsequent studies carried out in our group.
The first copper(I)-complexed trefoil knot could be crystallised and the crystals obtained were subjected to X-ray diffraction [14]. The structure obtained is shown on Fig. 10. Remarkably, the compound seems to undergo spontaneous resolution since only one enantiomer is found in a given crystal. This property seems to be somewhat general within the series of copper(I)-complexed knots made in Strasbourg. Among the few X-ray structures available for these complexes, only one shows that the compound crystallised as a racemate and not as a conglomerate of enantiomerically pure crystals [16].

X-ray structure of the original trefoil knot prepared in 1989 and crystallised in 1990 [14]. The knotted macrocycle is an 84-membered ring. The copper(I) atoms are green, the nitrogen and oxygen atoms are blue and red respectively. The linker between the two 1,10-phenanthroline nuclei of each strand is a (CH2)4 fragment. This linker is not very well adapted to the formation of a double stranded helix. In contrast, the use of a 1,3-phenylene spacer leads to quantitative formation of the double helix and, subsequently, to the formation of the trefoil knot in a very acceptable yield, as shown in Fig. 12.
Interestingly, the work of Vögtle and co-workers on the templated synthesis of [2]catenanes based on hydrogen bonds led to a very efficient preparation of a molecular knot [15]. This impressive synthetic achievement was probably not totally predicted by the authors themselves but the result is that another novel and really preparative method is now available.
Where will the chemistry of synthetic knots lead us? Today, it is of course difficult to know whether practical applications will be found, although one could easily imagine that polymers containing knotted fragments could be interesting organic materials or that knotted compounds able to interact in a specific way with DNA could display new biological properties. It remains that the field is still fascinating from a purely fundamental viewpoint. The challenge of making nontrivial prime knots beyond the trefoil knot is certainly worth considering, although when looking at the beautiful but very complex knots of Fig. 6, one can foresee great chemical difficulties. Obviously, chirality is an essential property in molecular chemistry, and knots are exciting systems in this context [15,16]. With a touch of fantasy, it could be conceived that some of the chemical processes for which chirality is crucial (enantioselection of substrates, asymmetric induction and catalysis, cholesteric phases and ferroelectric liquid crystals molecular materials for non linear optics etc.) could one day use enantiomerically pure knots. The future of molecular knots will, to a large extent, be determined by their accessibility and, even if the transition metal-templated strategy and the hydrogen bond-approach [15] represent interesting synthetic achievements, there is still a long way to go before molecular knots can be made at a preparative scale compatible with industrial applications.
4 Molecular machines based on catenanes and rotaxanes
A particularly promising area is that of synthetic molecular machines and motors [17]. In recent years, several spectacular examples of molecular machines leading to real devices have been proposed, based either on interlocking systems or on non-interlocking molecules [18]. In parallel, more and more sophisticated molecular machines have been reported, frequently based on multicomponent rotaxanes. Particularly noteworthy are the muscle-like compounds reported by two groups [19,20] as well as a molecular elevator [21], illustrating the complexity that dynamic threaded systems can reach.
One of the prototypical systems is a bistable catenane whose motions are triggered by an electrochemical signal. The compound and its various forms are represented in Fig. 13 [18a]. Copper is particularly well adapted to the design of molecular machines since its two oxidation states have distinct stereo-electronic requirements: whereas copper(I) is fully satisfied in a 4-coordinate (tetrahedral) geometry, copper(II) requires more ligands in its coordination sphere. A 5-coordinate situation is more adapted to the divalent state, as illustrated on Fig. 1, Cu(II) being coordinated to both a 1,10-phenanthroline ligand and a 2,2′:6’2″-terpyridine.

The prototypical bistable copper-complexed catenane [18a]. The compound undergoes a complete metamorphosis by oxidising Cu(I) or reducing Cu(II). The process is quantitative but slow.
In the course of the last 15 years, the response times of the various molecular machines made in Strasbourg have been considerably shortened. This is true for molecular “shuttles”, undergoing a translation motion, or pirouetting systems, experiencing a 180° rotation of a given fragment [22]. The fastest system is a rotaxane, able to undergo a “pirouetting” motion under the action of the same redox signal as for the catenane (CuII/CuI) and whose axis incorporates a non sterically hindering chelate of the 2,2’-bipyridine type [22a]. Nowadays, the motions are several order of magnitude faster than those of the first molecular machines made in our group several years ago. The two forms of this fast-moving [2]rotaxanes are represented in Fig. 14.

Electrochemically induced pirouetting of the ring in a fast-moving [2]rotaxane; the bidentate chelate and the tridentate fragment are alternatively coordinated to the copper centre. The system is able to switch on the micro- to millisecond time scale, even at low temperature (−40 °C) in DMF. As for all the copper-based dynamic catenanes and rotaxanes investigated, the unstable 5-coordinate copper(I) form is much faster to rearrange than the other thermodynamically unstable species generated in the cycle, namely the 4-coordinate copper(II) complex. This can be explained by the slower decoordination rate expected for a divalent metal than for its monovalent form.
About 8 years ago, our group has embarked in a project consisting in depositing our molecular machines on electrode surfaces so as to fabricate real devices. This approach is indeed promising in terms of functional materials and electromechanically responsive surfaces. Unfortunately, the project has been met with very limited success. Several potentially pirouetting [2]rotaxanes have been grafted on gold electrodes, either using thiolates or thioethers as anchoring groups. Whereas these compounds were shown to undergo relatively fast motions in solution after oxidising the tetrahedral Cu(I) form or by reducing the 5-coordinate Cu(II) complex obtained after rearrangement of the oxidised tetrahedral state, none of these complexes could be set in motion once they were deposited onto the electrode surface. In particular, a given [2]rotaxane, containing a non sterically hindering chelate in the axis, undergoes fast electrochemically induced pirouetting in CH2Cl2/CH3CN (1:9) with 0.1 M Bu4NBF4 as supporting electrolyte (Pt working electrode) [22c] but, to our surprise, it is totally inert to rearrangement once grafted on gold.
5 Two-dimensional interlocking arrays
In recent years, our group has also proposed transition metal-based strategies for making two-dimensional interlocking and threaded arrays [23,24]. Large cyclic assemblies containing several copper(I) centres could be prepared which open the gate to controlled dynamic two-dimensional systems and membrane-like structures consisting of multiple catenanes and rotaxanes. Two general families of such systems are depicted in Fig. 15.

Two-dimensional interlocking arrays built via the copper(I)-template strategy. The “gathering and threading effect” of Cu(I) leads to the quantitative formation of the pseudorotaxane tetramers (A) [23] or (B) [24] from the corresponding organic fragments and stoichiometric amounts of copper(I).
The quantitative synthesis of a four-copper(I) [4]pseudorotaxane made of two bis-macrocycles and two rigid rods threading the cycles is depicted in Figs. 16 and 17. The same compound can also be regarded as the cyclic tetramer of a [2]rotaxane.
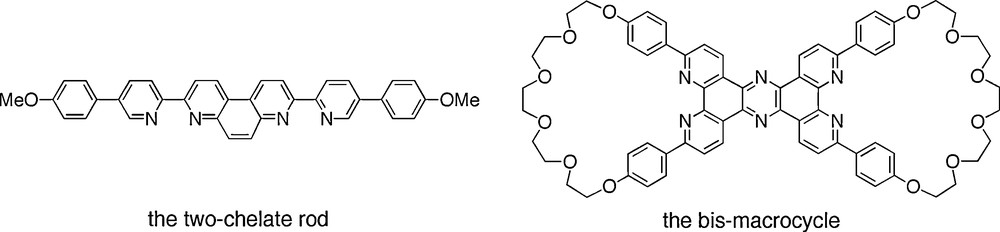
The organic fragments used for preparing a compound similar to (B) of Fig. 15.

The threading reaction, leading to the desired [4]pseudorotaxane in almost quantitative yield [24].
The X-ray structure of the four-copper(I) centre [4]pseudorotaxane of Fig. 17 was recently solved by Kari Rissanen and co-workers (Finland) [25]. It is shown in Fig. 18.

X-ray structure of the [2]pseudorotaxane. The red dots of the Scheme (right) represent the four copper(I) atoms [25].
6 A [3]rotaxane acting as an adjustable receptor: towards a molecular “press”
The role of porphyrin-containing catenanes and rotaxanes is still limited as far as the fields of molecular receptors and machines are concerned but these families of molecules are certainly promised a bright future within these research areas.
In the course of the last few years, our group has been interested in trying to construct a new molecular machine able to function as a “press” [26]. The principle and the role of the various components are explained in a schematic fashion in Fig. 19. Essential is the function of two porphyrinic plates, able to glide along the “track” which threads the rings to which they are firmly and rigidly attached. Starting from the metal-free [3]rotaxane represented on the bottom line, metalation of the coordination sites belonging to both the rod and the rings will induce a translation motion of the threaded rings: The two porphyrinic plates will come to closer proximity with respect to one another. As a consequence, a substrate trapped between these two plates will be compressed and, possibly, expelled from the rotaxane receptor. For the moment, the molecular system made is still primitive and functions more as an adjustable receptor than as a real molecular press. We hope that by controlling in a better way the geometrical properties of the compound, we will be able to elaborate an efficient molecular press. For space reasons, we cannot discuss the synthetic work carried out along this difficult and ambitious project.

(a) Schematic representation of a dicopper(I) rotaxane complex, in which the porphyrin-porphyrin distance is imposed by the geometry of the central dinucleating unit (represented by a double half of a circle symbol). (b) Principle of the adaptable [3]rotaxane receptor. The two rings threaded by the rotaxane axis (called “m-30” since they are 30-membered rings similar to traditional rings extensively used in our team) are rigidly attached to a Zn-porphyrin (blue moieties); they can glide along the axis so as to allow the receptor to adjust the Zn–Zn distance and thus to fit the dimensions of the L–L ditopic substrate. L is an organic fragment able to coordinate the Zn atom of the porphyrins.
The bisporphyrinic [3]rotaxane behaves as an efficient receptor towards guests of the type py-Z-py (py: 4-pyridyl; Z: spacer). Moreover, the ability of the two rings to glide freely along the axis onto which they are threaded permits the distance between the binding sites borne by the rings to be varied over a wide range, thus allowing guests of very different lengths to be complexed between these two sites. The principle of the recognition process and of the ability of the receptor to adapt to the guest is represented in Fig. 19.
The free [3]rotaxane 2 was obtained from a multistep procedure from various organic fragments [27]. The last steps involve: (i) a double-threading reaction of an unstoppered coordinating filament through the two coordinating rings, this process being driven by coordination to two copper(I) atoms; followed by (ii) a double-stoppering reaction allowing to attach the two terminal stoppers at the ends of the long thread. The compounds obtained are represented in Fig. 20 as well as the demetalation reaction leading to the free [3]rotaxane from the copper(I)-complexed precursor rotaxane.

Chemical structure of the copper(I)-complexed [3]rotaxane 1.2PF6 and of the free [3]rotaxane 2 obtained by demetalation of 12+ (the R groups represent 3,5-di-tert-butylphenyl substituents).
The synthesis of the constitutive elements of 12+ and 2 has recently been reported [25,28]. The core of the axis contains a 3,8-di(4-pyridyl)-4,7-phenanthroline motif, providing the axis with two rigidly connected bidentate chelates of the 2,2′-bipyridine family. The X-ray structure of an iridium(III) complex [29] of the coordinating central core indicates that the distance between the two complexed metal centres should be around 8-8.5 Å, in agreement with CPK models.
In order to verify that 2 can accommodate substrates of markedly different geometries, coordination of the two bispyridyl compounds 3 and 4 (Fig. 21) was studied. The substrate 3 is obviously geometrically very different from 4 since, in the latter substrate, the pyridyl groups are directly connected to one another through their four positions.

The two bispyridyl substrates 3 and 4.
The stability constants of the complexes , , and were determined by UV/Vis spectroscopic measurements. Their values, determined in toluene, are as follows:
The two latter values reflect both the adaptability of the [3]rotaxane host 2 and the respective basicity of guests 3 and 4. Obviously, 2 can adjust its geometry to the complexed guest. Although receptor 1.2PF6 has also some degree of adaptability, its corresponding association constants are significantly different from those of the free rotaxane. The long aromatic spacers between the porphyrin nuclei and the rings can easily be distorted. Nevertheless, coordination of 3 to the two zinc porphyrins of 12+ has to induce pronounced distortions of the receptor itself. Indeed, if the conformation of 12+ was frozen in the most stable one, as obtained by simulation, it would be virtually impossible to insert the substrate in between the two porphyrinic plates.
The two-porphyrin [3]rotaxane 2 represents the first example of an adaptable receptor based on interlocking or threaded compounds. The centre-to-centre distance between the two porphyrinic plates can vary substantially, roughly from 10 to 80 Å. The order of magnitude of the binding constants (about 106–107 M−1) underlines a cooperative effect between both porphyrins of the two receptor rotaxanes 12+ and 2. The analogy between the present system and a molecular press based on two mobile plates whose distance can be controlled from the outside is striking: the compression process is triggered by coordination of the two copper(I) atoms to the various coordination sites of the system, bringing the two plates together. The respective values of the association constants indicates that the longer substrate 3 is indeed compressed when interacting with 12+ whereas it is better accommodated in the free [3]rotaxane 2. Whereas formation of a complex with the short substrate 4 is favoured when copper(I) is introduced in the system, the opposite is observed for the longer substrate 3, in spite of the formation of a more rigid and organised host. The complexes are represented in Figs. 22 and 23. The stability constants are also indicated in the figures.

Schematic representation of the complexes formed between dipyridyl substrates and the free [3]rotaxane.

By bringing the two porphyrinic plates nearer to one another, the complex formed with the long substrate is destabilised whereas the complex with the shorter guest is stabilised.
7 Conclusion
It is still not sure whether the fields of catenanes, rotaxanes, knots and molecular machines will lead to applications in a short-term prospective, although spectacular results have been obtained in the course of the last few years in relation to information storage and processing at the molecular level [30]. From a purely scientific viewpoint, the field of molecular machines is particularly challenging and motivating: the fabrication of dynamic molecular systems, with precisely designed dynamic properties, is still in its infancy and will certainly experience a rapid development during the next decades. Besides potential practical applications, the elaboration of topologically nontrivial molecules has led to the discovery of new template principles, based on the three-dimensional arrangement of given organic fragments around a transition metal centre (mostly, copper(I)). Such an approach can be considered as a new synthetic method and presents an obvious character of generality. It has in particular allowed several groups to make and study fascinating compounds displaying highly novel topological properties in conjunction with new chemical and physical features as well as an indisputable aesthetic attractiveness.
Acknowledgements
We thank all the motivated and talented researchers who contributed to the work discussed in the present review. We are also grateful to the CNRS, to the Agence nationale de la recherche and to the European Commission for their constant support.