1 Introduction
Sea buckthorn (SB) is a plant that grows widely in various regions of Asia, Europe, and North America. All parts of the plant are considered to be a good source of a large number of bioactive substances, which have proved some medicinal and nutritional properties [1,2]. Sea buckthorn berries are orange/red in color, 10–15 mm in diameter. The berries are rich in vitamins, minerals and antioxidant components, including ascorbic acid, tocopherols, polyphenols and carotenoids [3,4]. These fruits are richer in β-carotene than carrots. Sea buckthorn leaves are small and narrow, 2–6 cm in length. Leaves are very rich in polyphenolic compounds and are reported to have antioxidant properties [5]. The major flavonoids in the extract of sea buckthorn are: catechin (CA), quercetin (QU), kaempferol (KA) and isorhamnetin (IS) [1]. These flavones have similar molecular structures, as shown in Fig. 1.
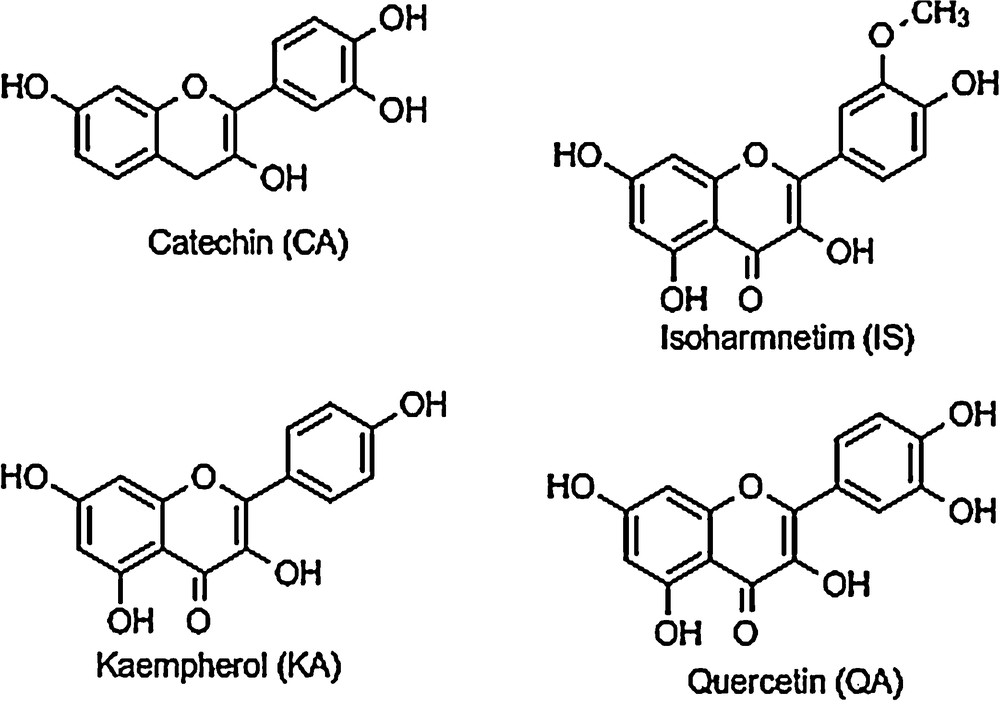
Chemical structures of flavonoids present in sea buckthorn extract.
Nanostructured lipid carriers (NLCs) based on a mixture of solid and liquid lipids are a new type of lipid nanoparticles, offering the advantage of having improved drug-loading capacity and release properties. There are several methods to produce nanostructured lipid carriers (NLCs), such as solvent evaporation in oil, in water microemulsion systems [6,7], solvent diffusion technique [8], high-pressure homogenization (HPH) technique [9,10], oil in water microemulsion technique [11], ultrasonic technique [12]. However, in comparison with other methods, the high shear homogenization (HSH) technique shows many advantages. It is a versatile technique that avoids the use of organic solvents and excessive energy or of other methods. It requires also a short production time.
The main objective of the present work was to obtain NLCs systems by the melt emulsification method coupled with high shear homogenization (HSH), with a view to the encapsulation of sea buckthorn extract (SBE) into the appropriate lipidic matrix with improved antioxidant and antimicrobial properties, with the purpose of developing potential applications as an efficient antioxidant delivery system. The choice of the lipid matrix and of the type of surfactant (with pharmacological use) is essential for obtaining safe and stable nanocarriers [13,14]. There exists a lot of plants that contain bioactive compounds with therapeutic effects in the treatment of various diseases. For this reason, three types of vegetable oils were used: grape seed oil (GSO), sea buckthorn oil (SBO), and St. John's wort oil (HPO) as liquid components of nanostructured lipid nanocarriers. They are known as efficient antioxidants [1,15], acting also as therapeutic agents that can prevent cardiovascular diseases, diabetes, and cancer [16]. The developed NLCs were characterized from the physicochemical point of view: average particle size, zeta potential, morphological characteristics, loading capacity. Therefore, SBE-loaded NLCs prepared with these three vegetable oils could be used as food supplements and could be considered as interesting alternatives to conventional antioxidant formulations.
2 Experimental part
2.1 Materials
Polyethylene glycerol sorbitan monolaurate (Tw20), polyethylene glycol sorbitan monooleate (Tw80), were obtained from Merck (Germany). Synperonic PE/F68 (block copolymer of polyethylene and polypropylene glycol), l-α-phosphatidylcholine, (lecithin) and tris[hydroxymethyl] aminomethane (luminol) were purchased from Sigma Aldrich Chemie GmbH (Munich, Germany). n-Hexadecyl palmitate (CP) 95% was purchased from Acros Organics (USA), while glycerol stearate (GS) was supplied by Cognis GmbH. Grape seed oil was purchased from S.C. Manicos SRL (Romania). Sea buckthorn oil and St. John's wort oil (Hypericum perforatum oil) were provided by Hofigal (Romania). Spectroscopic-quality chloroform (Fluka) and ethanol (Sigma Aldrich) were used as solvents. The sea buckthorn extract was obtained by us using the procedure described in a paper under preparation.
2.2 Preparation of NLCs
NLCs were prepared by a modified HSH method. This method was already described in a previous study [17,18]. An aqueous phase containing a surfactant mixture of Tw20 or, separately, Tw80:SynperonicF68:lecithin with a mass ratio of 1:0.25:0.25, was heated at 85 °C. The lipid-phase mixture, consisting of CP and GS either with GSO, SBO or with HPO in the weight ratio of 35:35:30, was heated at the same temperature for 30 min. Various amounts of SBE were added in the lipid phase to form a clear molten solution. Before mixing the two phases, the aqueous phase was stirred at high speed for 2 min at 15,000 rpm. By adding gradually the lipid phase into the aqueous one, different NLCs pre-emulsions were obtained (Table 1). After stirring 1 h at 85 °C, the pre-emulsions were processed by HSH with a Lab High-Shear Homogenizer PRO250 apparatus, at 25,000 rpm for 10 min. The lipid dispersions were cooled down to room temperature under stirring. The water excess was removed by lyophilization, by using an Alpha 1-2 LD Freeze Drying System. The NLCs suspensions were frozen for 24 h at –25 °C, and then the samples were transferred into the freeze dryer at –55 °C for 72 h in order to obtain both unloaded NLCs and loaded SBE-NLCs powders.
Composition and physicochemical characterization of free nanostructured lipid carriers (NLCs) and sea buckthorn extract (SBE)-NLCs.
Sample | Composition* | Pdl ± SDs | ξ [mV] ± SDs | DSC characteristics | |||
SBE % w/w | Main surfactant | Oil type | C.I. | P.t./shoulder °C | |||
Free NLC1 | – | Tw20 | GSO | 0.190 ± 0.01 | –37.8 ± 1.87 | 63.85 | 49.1 |
NLC-SBE1 | 0.5 | Tw20 | GSO | 0.201 ± 0.009 | –60.5 ± 0.651 | 91.48 | 50.9 |
NLC-SBE2 | 1 | Tw20 | GSO | 0.204 ± 0.006 | –46 ± 0.854 | 85.48 | 49.5 |
Free NLC2 | – | Tw80 | GSO | 0.213 ± 0.012 | –33.9 ± 1.63 | – | – |
NLC-SBE3 | 0.5 | Tw80 | GSO | 0.257 ± 0.011 | –39.8 ± 1.44 | 63.11 | 45.6 |
NLC-SBE4 | 1 | Tw80 | GSO | 0.247 ± 0.012 | –47.7 ± 1.57 | 87.85 | 52 |
Free NLC3 | – | Tw20 | SBO | 0.208 ± 0.009 | –44.7 ± 0.819 | 76.05 | 50.5 |
NLC-SBE5 | 0.5 | Tw20 | SBO | 0.173 ± 0.016 | –52.2 ± 1.08 | 90.83 | 49.6 |
NLC-SBE6 | 1 | Tw20 | SBO | 0.220 ± 0.015 | –60.2 ± 1.48 | 80.26 | 49.6 |
Free NLC4 | – | Tw80 | SBO | 0.221 ± 0.013 | –40.5 ± 0.473 | – | – |
NLC-SBE7 | 0.5 | Tw80 | SBO | 0.259 ± 0.021 | –42.1 ± 0.451 | 84.12 | 48.8 |
NLC-SBE8 | 1 | Tw80 | SBO | 0.252 ± 0.011 | –39.2 ± 0.85 | 87.15 | 48.7 |
Free NLC5 | – | Tw20 | HPO | 0.214 ± 0.005 | –55.3 ± 0.721 | – | – |
NLC-SBE9 | 0.5 | Tw20 | HPO | 0.203 ± 0.008 | –51.8 ± 1.42 | 90.73 | 50.6/39.7 |
NLC-SBE10 | 1 | Tw20 | HPO | 0.228 ± 0.007 | –48.1 ± 0.929 | 91.70 | 51.7/39.8 |
Free NLC6 | – | Tw80 | HPO | 0.227 ± 0.011 | –57.9 ± 0.361 | 82.86 | 49 |
NLC-SBE11 | 0.5 | Tw80 | HPO | 0.226 ± 0.013 | –59 ± 3.12 | 95.16 | 52/42 |
NLC-SBE12 | 1 | Tw80 | HPO | 0.257 ± 0.011 | –39.8 ± 1.44 | 95.30 | 50.9/45.3 |
* All NLCs samples were prepared with 10% (w/w) lipid mixtures, in a ratio of CP:GS:GSO/SBO/HPO = 1.16:1.16:1.
2.3 Particle size measurements
Particle size analysis was performed immediately after NLCs preparation and after 6 months of storage, by dynamic light scattering (DLS), with a Zetasizer Nano ZS (Malvern Instruments Ltd.). The particle average size (Zave) and the polydispersity index (PI) values were obtained as an average of three measurements at 25 °C. The light incidence angle was fixed at 90°. Prior to the measurements, all the samples were diluted with deionized water in order to obtain an adequate scattering intensity.
2.4 Zeta potential measurements
The zeta potential (ZP), reflecting the electric charge on the particle surface and indicating the physical stability of the colloidal systems, was measured by determining the electrophoretic mobility using the zetasizer Nano ZS. The measurements were performed in deionized water and the conductivity was adjusted to 50 μS/cm with a solution of sodium chloride (0.9% w/v). All measurements were performed in triplicate at a temperature of 25 °C.
2.5 Transmission electron microscopy (TEM)
The morphology of lyophilized SBE-NLCs was determined using a transmission electron microscope (Philips 208 S). The studied NLCs dispersion was diluted with deionized water (1:50) and a drop of the NLCs solution was placed onto a carbon-coated copper grid and dried at room temperature for 1 h before examination.
2.6 UV-VIS analysis
The UV-VIS-NIR spectra of lyophilized SBE-NLCs powders in MgO pellets were recorded in the 220–2200-nm wavelength range, using a Jasco double-beam V670 spectrophotometer (the diffuse reflectance analysis was performed with the ILN–725device equipped with an integrating sphere).
2.7 Differential scanning calorimetry (DSC) analysis
The degree of crystallinity and polymorphism of the NLCs was evaluated by thermal analysis using a DSC 204 F1 apparatus (Netzsch). Approximately 10 mg of lipid dispersion were accurately weighted into an aluminum pan and sealed hermetically. An empty aluminum pan was used as a reference. The samples were heated in the 25–100 °C temperature range at a heating rate of 5 °C/min. The crystallinity index (Ci) was calculated using Eq. (1):
(1) |
2.8 Antioxidant activity
The in vitro antioxidant activity of unloaded NLCs and loaded SBE-NLCs was determined and compared with that of SBE by the chemiluminescence method (CL) using a Chemiluminometer Turner Design TD 20/20, USA. For this purpose, the luminol + H2O2 system was used as a generator one, in a Tris–HCl buffer solution (pH = 8.6). The antioxidant activity of ethanol solutions of lyophilized unloaded NLCs and loaded SBE-NLCs was calculated using Eq. (2):
(2) |
2.9 Antibacterial activity
The antibacterial activity of NLCs was tested against Escherichia coli K 12-MG1655. It was measured by using the agar well diffusion method [19]. The bacterial strains were grown on Luria Bertani Agar (LBA) plates at 37 °C with the following average composition: peptone, 10 g/L; yeast extract 5 g/L, NaCl 5 g/L, and agar 20 g/L. The stock culture was maintained at 4 °C.
Sterile LBA plates were prepared by pouring the sterilized media on sterile Petri dishes under aseptic conditions. One milliliter of the test organism was spread on agar plates. Using a sterile 6-mm Durham tube, the wells were made in the same way for all studied samples. They were inoculated with 50 μL of NLCs dispersion. Each plate carried a blank well by adding solvent (ethanol) alone to serve as a negative control. All plates containing bacteria were incubated at 37 °C for 24 h.
The antibacterial activity of the microorganisms with NLCs was determined by measuring the size of the inhibition zone (IZ, mm) as a clear, distinct zone of inhibition surrounding the agar wells. The values smaller than 8 mm were considered as being not active against the microorganisms. All experiments were performed in triplicate. The obtained results are shown in Fig. 7.
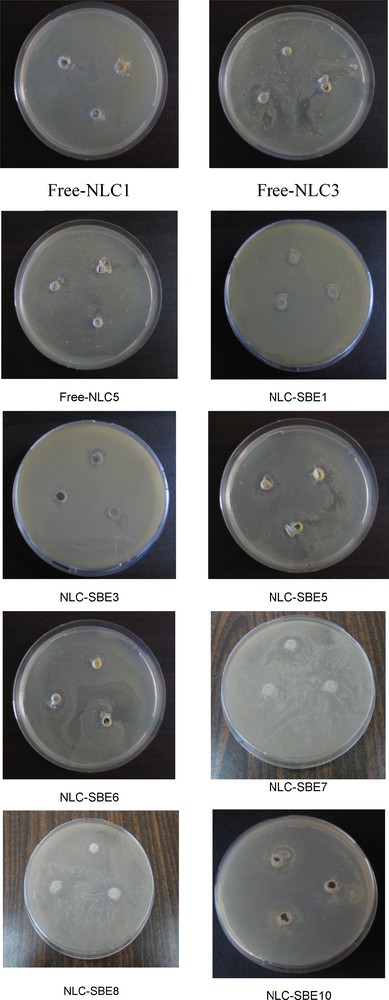
Antibacterial activity of sea buckthorn extract (SBE)-nanostructured lipid carriers (NLCs) against Escherichia coli (color online).
3 Results and discussion
3.1 Particle size analysis
In order to find the optimum conditions for obtaining NLCs with a monomodal profile and high antioxidant properties, a series of comparative experiments have been performed by using two kinds of non-ionic surfactants and a mixture of lechitine with Synperonic F68. In the optimized Tw20- or Tw80-containing systems, the influence of sea buckthorn extract concentration was measured by the dynamic light scattering (DLS) technique in order to determine its effect on the size distribution of the lipid nanoparticles.
The particle size parameters (average particle size, its distribution and the polydispersity index (Pdl) of developed NLCs dispersions) have been evaluated by DLS measurements and are shown in Fig. 2. This method explores the dependence of the diffraction angle on the particle radius [20]. The data were collected on the very day when the samples were prepared. For comparison purpose, similar measurements were performed on unloaded lipid nanoparticles as well. The size of all the studied SBE-NLCs ranges between 167 and 243 nm. The measured characteristics of all unloaded and loaded-NLCs samples are listed in Table 1.

Dimensional analysis of nanostructured lipid carriers (NLCs)-sea buckthorn extract (SBE) systems prepared with different oils: GSO (a), SBO (b), and HPO (c) (color online).
By comparing the effectiveness of the three types of vegetable oils (GSO, SBO, and HPO), it was observed that the lipid matrix prepared with GSO gives the smallest average particle diameter size for the free lipid matrix, Zav = 125.6 nm, while for the lipid matrices prepared with HPO and SBO, higher values were obtained, Zav = 142.2 nm and Zav = 156 nm, respectively.
Referring to the effect of the surfactant used, the smallest NLCs were obtained (Zav = 174.4 nm and a Pdl of 0.203) when Tw20 was used as the main non-ionic surfactant and with an initial concentration of 0.5% SBE, while the largest NLCs (Zav = 243.1 nm and a Pdl of 0.252) were got with Tw80 at a concentration of 1% SBE.
Concerning the influence of SBE concentration on the average particle diameter of encapsulated NLCs, one observes it increases for NLCs loaded with SBE. Moreover, the average particle diameter increases when the initial concentration of SBE increases from 0.5% to 1%.
The results of polydisperity index studies for NLCs containing 0.5% and 1% SBE, show the following aspects:
- • for the same amount of SBE (0.5% or 1%), the Pdl is almost the same within the experimental accuracy;
- • the Pdl increases with increasing the SBE concentration in NLCs.
3.2 Dispersion stability
The measurement of zeta potential allows us to predict the thermodynamic stability of colloidal dispersions. Zeta potential is the electrostatic potential at the plane of shear and reflects the amount of electric charge on the particle surface. It represents an important component in the evaluation of the physical stability of colloidal dispersions [21–23].
Generally, the stability analysis of studied lipid nanodispersions reveals that all NLCs exhibit a negative electrokinetic potential as given in Table 1, with values ranging between –30 mV and –60 mV. The lipid nanoparticles loaded with SBE and prepared with Tw20 as the main surfactant show a better physical stability than those prepared with Tw80. Actually, these zeta potential values (cf. Table 1) show that all the NLCs dispersions obtained using the three vegetable liquid oils and a modified HSH method are physically stable systems. Moreover, the electrokinetic potential for the free-NLCs studied depends on the type of vegetable oil used. The highest value is obtained when using GSO, while the lowest is got for the NLCs containing HPO.
On the other hand, the higher values of electrokinetic potential of free NLCs compared with loaded SBE-NLCs could be associated with the incorporation of SBE into the lipid mixture. This leads to a rearrangement of the lipid network and/or coating of surfactants, thus to a surface modification, as it will be further confirmed by differential scanning calorimetry (DSC) and transmission electron microscopy (TEM) measurements.
Indeed, the electronegative values of the electrokinetic potential show that the aqueous dispersions are physically stable systems with a strong repulsion tendency between particles, resulting in a low probability of NLCs aggregation.
In order to confirm the conclusions concerning zeta potential values and to check the temporal stability of the loaded and unloaded NLCs, the size distribution parameters and zeta potential were also measured 6 months after sample preparation. They were kept at a temperature of 4 °C and the results were compared with those obtained on the day of preparation. An increase of the size distribution by 10–15 nm has been observed as compared to the initial values. Similarly, a slight increase of zeta potential by ca 10 mV was also registered, thus supporting the NLCs stability during storage.
3.3 Transmission electron microscopy (TEM)
A representative TEM image of sea-buckthorn-extract-loaded NLC is shown in Fig. 3. One can see that the particles are endowed with nanometer-size spherical shapes, with a visible shell of surfactants surrounding the lypophile core. The inner part of nanoparticles reveals an amorphous structure. It is known from the literature that drug incorporation into nanoparticles always leads to their size increase as compared to the free-NLCs [24,25]. Based on this assumption, the encapsulation of SBE into the lipid matrix increases the size of the nanoparticles, which shows that the SBE indeed was loaded into NLCs, in accordance with the DLS measurements (Fig. 2).

Transmission electron microscopy image of sea buckthorn extract (SBE)-nanostructured lipid carriers (NLC2) prepared with GSO.
The mechanism of SBE encapsulation is rather complicated, as a result of the complex structure of the selective plant extracts, containing both lipophilic and hydrophilic components. According to GC–MS analyses (results not presented here), SBE contains major components hydrophilic derivatives such as inositol (42%) with glicopirazon (12%) as a representative compound, and also lipophilic components, such as sitosterol (16%). It is supposed that sitosterol can be easily incorporated into the lipid core, while inositol, due to the presence of multiple OH groups, will be distributed towards the surfactant shell, as can be seen in Fig. 3, as smaller particles surrounding the NLCs core.
By corroborating the results provided by DLS and TEM measurements, one can conclude that SBE-loaded NLCs with good physical stability are obtained by using the HSH method and that particle aggregation during storage is unlikely to occur.
3.4 Electronic spectra
The electronic spectra were registered in reflectance mode on powders for all free and encapsulated NLCs obtained. Their interpretation is based on UV–VIS–NIR transitions of the corresponding pure compounds identified by GC–MS as major components present in all three types of oils (GSO, SBO, HPO) and surfactants used (Tw20, Tw80).
To demonstrate the encapsulation of active compounds into the lipidic matrix, we have measured the electronic spectra (cf. Fig. 4a–e) in various samples by comparing free NLCs and SBE-loaded NLCs. Thus, for free NLCs containing GSO within the lipid matrix, one may recognize the peak at 248 nm belonging to surfactant Tw20 and the one at 268 nm specific to the major components of GSO (Fig. 4a: oleic, linoleic acids, tocopherol). The band specific to chinonic compounds of SBE as bioactive components at 456 nm (Figs. 4a–e) is clearly recognized in encapsulated NLC-SBE1 and NLC-SBE2 with intensities proportional to their content, 0.5% and 1% respectively (Fig. 4a). A similar behavior is also observed for NLCs encapsulated with SBE and Tw80 as the surfactant (Fig. 4b).

UV–Vis absorption spectra of nanostructured lipid carriers (NLCs) loaded with sea buckthorn extract (SBE): a: NLCs prepared with GSO and Tw20; b: NLCs prepared with GSO and Tw80; c: NLCs prepared with SBO and Tw20; d: NLCs prepared with SBO and Tw80; e: NLCs prepared with HPO and Tw20; f: NLCs prepared with HPO and Tw80 (color online).
When SBE is used both as a component of lipid matrix and as an encapsulated active compound, the electronic spectra (Fig. 4c, d) contain in the UV domain bands of various intensities at 242–248 nm and at 300–314 nm. The first of them is dominant in SBO due to the highest content in oil acids, while the second band is dominant in SBE due to a higher content in polyphenolic compounds. Moreover, apart from the central large band at 438–466 nm specific to chinoidic structures present both in oil and extract, another sharp band at 664 nm is evidenced in all the samples.
The encapsulation of SBE 0.5% into the lipid matrix is clearly evidenced by more intensive bands at 248, 304 and 444 nm, then those of free NLCs (Fig. 4c). An unusual effect is noticed when SBE 1% is used for encapsulation into the lipid matrix, when the band at 452 nm is drastically diminished. This behavior suggests the impossibility to incorporate higher amounts of bioactive compounds into the SBO lipid matrix. It seems that at higher contents of SBE, this contributes to expel SBO from the NLCs core. Actually, most of SBE remains outside NLCs, as it was evidenced in the TEM image in Fig. 3 ; after having been maintained for 2 h at 89 °C, it is degraded. A similar behavior is noticed when Tw80 is used as the surfactant.
In the samples prepared with HPO as a liquid lipid component, its specific contribution at 412 nm (Fig. 4e) in the spectra of free NLCs cannot be evidenced, since it has lower intensity than that of SBE. Therefore, the electronic spectra of encapsulated NLCs with SBE are similar to those of the previous lipid matrices. However, the intensities of the SBE band at 456 nm are almost identical when the concentration is doubled (0.5% and 1%). This behavior suggests that only ca. 0.5% of SBE is encapsulated. One may conclude that the first matrix containing GSO is favorable for SBE encapsulation as compared to that containing HPO. This is confirmed by the electronic spectra of similar NLCs prepared with Tw80 when the content in encapsulated SBE is lower in the NLC sample with 1% SBE than in the sample with 0.5% SBE (Fig. 4f).
3.5 DSC measurements
Since the lipidic core is subjected to a transition from a less ordered to a more ordered solid state during drug release, it is essential to verify the solid state and the polymorphism of the NLCs. In this respect, the DSC technique was used to investigate the polymorphism and the degree of crystallinity of the studied NLCs. DSC analysis is based on the fact that various lipid modifications possess different melting points and melting enthalpies [13,26]. In order to investigate different effects on the state of crystallization behavior of NLCs prepared by the HSH method, bulk materials, free-NLCs and SBE-NLCs were comparatively studied. The melting points correspond to the maximum of the heating curve. The thermograms of different SBE-NLCs are presented in Fig. 5. The recorded DSC parameters are given in Table 1.
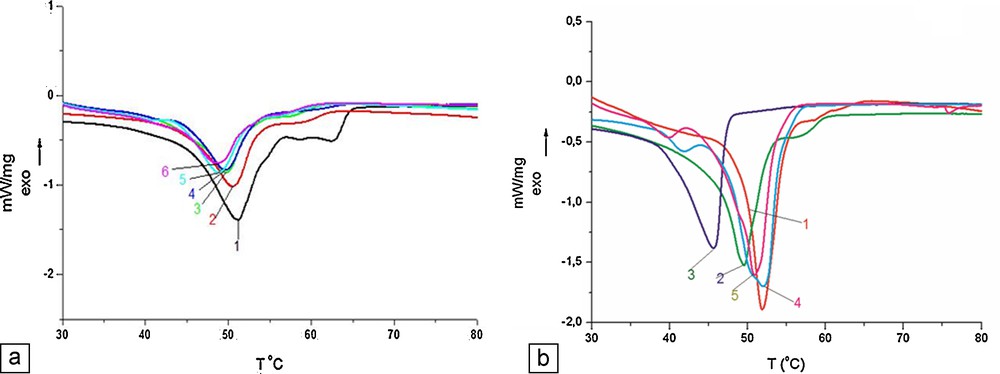
DSC curves for the lyophilized sea buckthorn extract (SBE)-nanostructured lipid carriers (NLCs), compared with free-NLCs and physical lipid mixture: a: NLCs prepared with SBO (1, bulk; 2, free NLC3; 3, NLC-SBE5; 4, NLC-SBE6; 5, NLC-SBE7; 6, NLC-SBE8); b: NLCs prepared with GSO (1, free NLC; 2, NLC-SBE1; 3, NLC-SBE2; 4, NLC-SBE3; 5, NLC-SBE4) (color online).
For the three bulk samples prepared with GSO, SBO and HPO, the melting points are similar, ranging between 51.0 and 51.2 °C, with shoulders between 62.4 and 62.6 °C due to the surfactants, while the corresponding enthalpy values are 153.7 J/g, 143 J/g and 140.7 J/g, respectively. As can be seen from Table 1, all free NLCs and SBE-NLCs showed a significant decrease in their melting temperature as compared with all three different bulks.
Fig. 5a shows the change in crystallinity highlighted by the thermal behavior of loaded SBE-NLCs prepared with two kinds of non-ionic surfactants (Tw20 and Tw80) and SBO as compared with those of the bulk lipid mixture and of free NLCs. The loaded NLCs prepared with Tw80 reveal a less ordered crystalline arrangement reflected by lower CI values. Its endothermic peak decreases in intensity as compared with that of free-NLCs. On the contrary, the loaded NLCs prepared with Tw20 show a lipid arrangement similar to that of free NLCs. These aspects prove a better accommodation of SBE in the lipid matrix formed with Tw80 than in those formed with Tw20.
Fig. 5b illustrates the thermal behavior of loaded SBE-NLCs prepared with two kinds of non-ionic surfactants and GSO as a lipid matrix. The shape of the DSC curves demonstrates that the crystallinity degree is different for each one of the studied samples. By comparing free-NLCs with SBE-loaded NLCs, one can observe that the incorporation of SBE into the lipid matrix leads to a decrease of the crystalline arrangement, highlighted by the decrease of the endothermal peak intensity of SBE-NLCs.
3.6 Antioxidant activity
The antioxidant properties were studied by the chemiluminescence method, which is an appropriate technique for measuring the presence of free oxygen radicals [27,28]. For comparative purposes, the solutions of free NLCs, SBE-NLCs, SBE, GSO, SBO, and HPO were exposed to a free radical generator system that releases free, high-energy intermediate radicals. In this context, the antioxidant activities of sea buckthorn both as oil and extract exhibit values above 80%, being higher for SBE than for SBO (Fig. 6a), in accordance with dominant polyphenolic components in extract as compared to the oil.
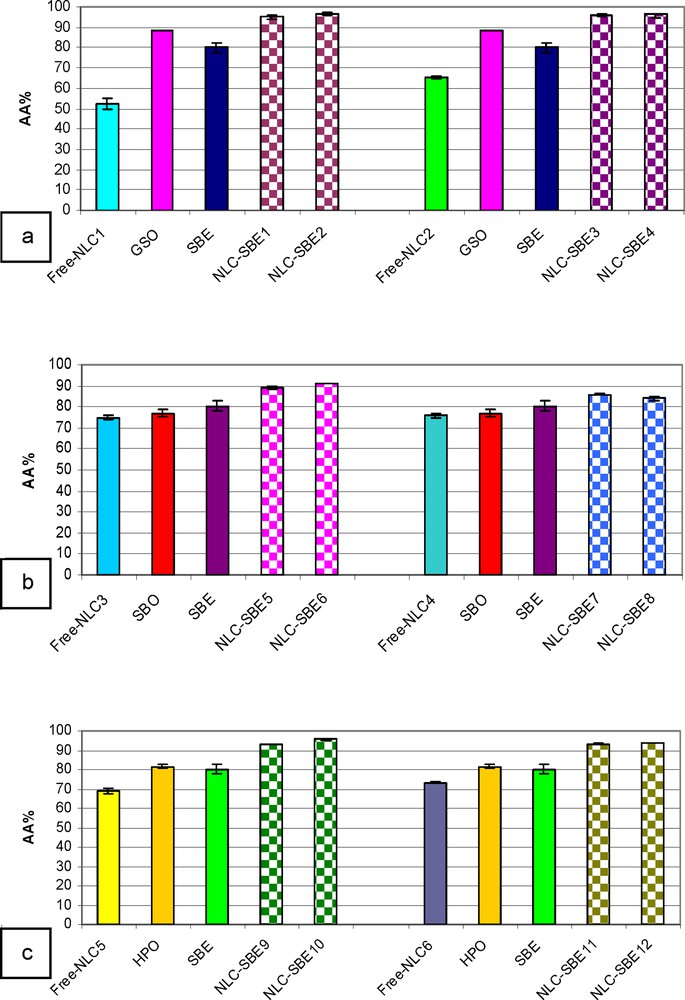
Antioxidant activity of SBE-nanostructured lipid carriers (NLCs), prepared with the following oils: GSO (a), SBO (b), and HPO (c) (color online).
In all the tested samples, the antioxidant activity of encapsulated sea buckthorn extract was significantly higher than that of SBE in bulk. The obtained SBE-NLCs have the ability to scavenge the free radicals between 95.01 and 96.50% for the systems prepared with GSO, between 84.19 and 90.87% in the case of SBE-NLCs prepared with SBO, and between 93.18 and 95.63% for the SBE-NLCs prepared with HPO. The lowest antioxidant activity obtained for the SBE-NLCs prepared with SBO can be explained by the fact that a part of SBO is expelled from the lipid matrix, leading to a rearrangement of the lipid network into a more ordered structure, as we have seen from DSC and TEM analyses.
Referring to the three oils investigated (Fig. 6a–c), their AA is increasing in the series SBO < HPO < GSO. This behavior is a result of the presence of highly antioxidant compounds in GSO, such as resveratrol, which is well known for its therapeutic properties [29].
When these oils are used as a lipid core within NLCs, their AA is drastically reduced, probably due to the inactivation of many OH groups as a result of encapsulation. These interactions are stronger in more reactive oils; this is why the AA is now increasing in the reverse order: GSO < HPO < SBO.
In case all NLCs are loaded with SBE, the AA is further enhanced as a result of a synergistic effect between the complex structural lipid matrix and the encapsulated bioactive SBE. The antioxidant activity of the loaded SBE-NLCs is much higher than that of SBE ethanolic solutions (Fig. 6a–c).
The use of the two surfactants Tw20 and Tw80, as well as of two concentrations of bioactive extract (0.5 and 1%), does not lead to significant differences, except for the lipid matrix containing SBO when AA is relatively lower. This is once again in perfect agreement with the conclusions drawn from electronic spectra, DLS, DSC and TEM measurements, thus confirming the loss of SBO at higher concentrations of SBE 1%.
To summarize, the observed differences in the antioxidant capacity of the analyzed samples depend on various factors, such as: the type of surfactant, the vegetable oil used, the concentration of the encapsulated extract, and the type of solid lipids used for the lipid matrix (CP and GS) which lead to the formation of free fatty acids or other free radicals than those present in our system. It is known from the literature that the saturated fatty acid undergoes a peroxidation process resulting in the formation of peroxy and hydroperoxides free radicals [30,31].
Another important factor contributing to an enhancement of antioxidant activity is provided by the synergy between lipids and the surfactant mixture.
3.7 Antibacterial properties
It is well known from the literature that vegetable oils can be a rich source of antimicrobial agents [32]. Therefore, we have tested the unloaded and loaded sea buckthorn extract nanostructured lipid carriers for their potential antibacterial activity against E. coli bacteria, which is accepted as an indicator of food products contaminations. All tested NLCs resist this bacteria. Additionally, some of the SBE-NLCs are highly effective against bacteria development.
The analysis of the antibacterial activity of the studied NLCs samples has shown its variation with sea buckthorn extract concentration and with the type of vegetable oil used. The best antibacterial activity against the tested bacteria was observed for SBE-loaded NLCs containing GSO, followed by the SBE-NLCs prepared with HPO. The smallest effect was registered in SBE-NLCs prepared with SBO. This last aspect is once again in good agreement with the conclusions drawn from the electronic spectra, DLS, DSC and TEM measurements, thus confirming the repel of SBO as discussed previously.
Moreover, it is interesting to note that the antibacterial activity of free NLCs follows the same order for the three oils in the case of antioxidant activity: SBO < HPO < GSO (numbers are shown in Fig. 7 and values are given in Table 2).
Inhibition zone diameter of nanostructured lipid carriers (NLCs) against Escherichia coli.
Sample | IZ for pathogenic microorganism, mm Escherichia coli |
S | 13.0 ± 0.17 |
SBE | 16 ± 0.34 |
Free NLC1 | 11.3 ± 0.11 |
NLC-SBE1 | 20.5 ± 0.50 |
NLC-SBE2 | 13.2 ± 0.20 |
Free NLC2 | – |
NLC-SBE3 | 21.0 ± 0.5 |
NLC-SBE4 | 13.16 ± 0.15 |
Free NLC3 | 19.7 ± 0.55 |
NLC-SBE5 | 17.3 ± 0.23 |
NLC–SBE6 | 24.3 ± 1.36 |
Free NLC4 | – |
NLC-SBE7 | 16.7 ± 0.41 |
NLC-SBE8 | 13.0 ± 0.17 |
Free NLC5 | 12.0 ± 0.2 |
NLC-SBE9 | 11.23 ± 0.25 |
NLC-SBE10 | 25.83 ± 1.04 |
Free NLC6 | – |
NLC-SBE11 | 18.5 ± 0.3 |
NLC-SBE12 | 20.5 ± 0.5 |
The bioactive extract sea buckthorn shows a good antibacterial activity against the control solution (EtOH), with an inhibition zone of 16.00 ± 0.34 mm as compared to 11.17 ± 0.28 mm. When SBE is encapsulated in these lipid cores, the best antibacterial activity is obtained for HPO in Tw20 with SBE of 1%.
It follows that the SBE is highly effective against E. coli. The antibacterial activity depends on the type of vegetable oil used.
The present study demonstrates that SBE encapsulated into various lipid nano-matrices is a highly efficient natural antioxidant that can be successfully used either as food supplement or as an antimicrobial agent in pharmaceutical industry.
4 Conclusion
The results obtained in this study indicate that the high-shear homogenization method is a versatile technique suitable for obtaining sea-buckthorn-extract-loaded NLCs with high antioxidant and antimicrobial properties. These combined activities are also due to the presence of three vegetable oils grape seed, sea buckthorn and H. perforatum oils as bioactive components of the lipidic matrix.
The developed SBE-loaded NLCs were characterized from the physicochemical point of view for their particle size, physical stability, and structural modification.
Most of NLCs dispersions produced by HSH are characterized by an average particle size below 200 nm with a good size distribution (Pdl < 0.25). Using Tw20 as the main non-ionic surfactant and a concentration of 0.5% SBE, smaller lipid nanoparticles were obtained.
The studied NLCs show also an excellent physical stability in time, as demonstrated by the measured zeta potential values, ranging from –33.9 mV to –60.5 mV.
Differential scanning calorimetry analysis has shown that the use of Tw80 for encapsulation of SBE in NLCs leads to a more disordered lipid network as compared with free NLCs.
The comparative studies of UV–Vis electronic spectra show that the most favorable lipid matrix for SBE incorporation is that obtained with grape seed oil and an SBE content not higher than 0.5%.
The evaluation of in vitro antioxidant properties has indicated an enhancement of the antioxidant activity for all SBE-NLCs studied when they are prepared with each one of the three types of vegetable oils: GSO, SBO and HPO as compared with free NLCs.
The antibacterial activity tests against E. coli show that most of SBE-loaded NLCs exhibits an inhibition zone higher than that for SBE in bulk.
Based on the results presented in this study related to the antioxidant and antibacterial activities of SBE-loaded NLCs prepared with the three vegetable oils, one can conclude that the new developed NLCs are potentially interesting for use as food supplement or antimicrobial agents in pharmacology. Such plant extracts may help to develop nanomedicines as efficient remedies against various human and animal pathogens. However, further clinical studies and testing of SBE-NLCs efficiency are needed.
Acknowledgements
The work has been funded by the Sectorial Operational Programme “Human Resources Development 2007–2013” of the Romanian Ministry of Labour, Family and Social Protection through Financial Agreements POSDRU/107/1.5/S/76903.