1 Introduction
Zeolites are referred to as ‘molecular sieves’. They are low-density, crystalline aluminosilicate materials that possess regular micropores with a dimension range of 0.3–2 nm [1]. 232 different zeolite framework types are currently known [2]. Depending on the structure type the zeolite framework composition can be varied to different extents. Thus the diversity of zeolitic materials is extremely large, from both structural and chemical points of view. The number, nature and distribution of the active centres are of paramount importance for the efficiency of a heterogeneous catalyst. Zeolite structures comprise channels and cages that make the crystalline frameworks accessible to foreign species. Thus they offer great advantages with respect to non-porous solids. The available surface for bulk materials depends on the particle size. Hence, the only option to increase the available surface is to decrease the size of the crystals and respectively increase the portion of the atoms that are on the crystal surfaces.
Open zeolite structures offer high accessibility of their atoms, which is combined with unrivalled shape selectivity. However, a serious drawback in zeolite-catalysed reactions is that reactants and products may face severe diffusion limitations that often lead to pore blocking and catalyst deactivation by coke formation. The efficiency of separation processes in zeolites is strongly influenced by intra-pore diffusion. A lot of work has been dedicated to the preparation of zeolites with enhanced accessibility to the micropore volume. A straightforward approach relies on the shortening of the diffusion path by decreasing the zeolite crystal size [3]. The impact of diffusion limitation is substantially reduced when nanocrystals with a size of below 50 nm are obtained. In such a case up to 20% of tetrahedrally (T) coordinated atoms can be located on the external surfaces [4]. There are several reviews addressing diverse aspects of nanosized zeolite preparation and their uses [5].
This feature article covers solely new developments in the synthesis of nanozeolites that provide ultra small nanocrystals of high quality. The decrease of zeolite crystal size to 10–15 nm leads to a considerable increase in their external surface areas, which in turn leads to the enhanced properties associated with the latter (adjustable surface charge, hydrophilicity/hydrophobicity, and high external surface activity). These surface properties provide new possibilities to explore adsorption and reaction of bulky molecules that cannot interact with the micropore volume of the zeolites.
The successful synthesis of ultra small nanoparticles raises also new challenges related with their shaping prior to applications in catalysis, sorption processes, and as membranes and films for optical purposes. This intermediate stage between the synthesis and the use of zeolites will be addressed in the present overview. Finally, the emerging applications of nanosized zeolite crystals will be reviewed.
2 Nanozeolite synthesis: particularities
A conventional zeolite synthesis yields crystals with a dimension of about 1 μm. At least one order of magnitude smaller particles are considered nano (<100 nm); however the impact of the particle size over the physicochemical properties become more pronounced when the crystallites are smaller than 50 nm and even below 30 nm. Similar to the conventional micron-sized crystals, the nanosized zeolites are synthesized under hydrothermal conditions in closed vessels and no reactants are added during the reactions. Thus after the conversion of the reactants in the system, the growth stops. In the synthesis of nanosized zeolites special attention is paid to the preparation of the initial precursor system in order to favour the nucleation process. The importance of the nucleation is due to the fact that the number of nuclei in the system determines the ultimate crystal size; thus an abundant nucleation leads to very small crystals while if the system yields a few viable nuclei the crystals formed are large.
Although different approaches have been explored to obtain nanosized zeolites, namely confined space [6], microreactor [7], microwave (MW) [8] and ultrasonic [9] radiation synthesis, conventional hydrothermal crystallization is mostly used. Hydrogels are used as precursors, but the way of preparation is particular in order to: i) favour the nucleation over the growth and ii) limit the aggregation between the growing crystals.
The first systems employed for the preparation of nanosized zeolites with a narrow particle size distribution were optically clear water suspensions, which contained only discrete gel particles [10]. The stabilization of a precursor suspension containing only discrete zeolite precursor particles requires specific conditions in each stage of the preparation. Firstly, the employed reactants and the temperature of mixing have to be properly selected. Furthermore, the alkali metal content has to be as low as possible in order to avoid aggregation between the particles. The absence of alkali metal cations is compensated by abundant amounts of tetraalkylammonium (TAA) hydroxides that keep the basicity of the system high and act as structure directing agents (SDAs). The homogenisation of the reactants is gentle to avoid the aggregation of precursor particles. Thus a water clear suspension containing particles that are uniform in size is stabilized. Usually the system used is diluted to avoid aggregation between the growing crystals. The crystallization temperature is typically lower than the one traditionally used for the synthesis of a particular zeolite, which favours nucleation over growth. Although not completely homogeneous, these systems provide the necessary conditions for uniform nucleation and crystal growth. Consequently the nanosized particles obtained are uniform in size and the agglomeration of the particles is limited. Upon heating, the amorphous precursor particles convert into nanosized zeolite crystals. The final appearance of the suspension depends on the size and the concentration of particles, and it varies between turbid and milky white. This method of synthesis is efficient for laboratory needs; the crystals are uniform in size and can be stabilized in the colloidal form. The yield, however, is typically low. This is due to low conversion of the silica/alumina precursor due to the mild conditions and limited use of alkali metals.
A number of fundamental studies were performed in order to shed more light into the intimate mechanism of precursor conversion and thus to optimize the synthesis of nanosized zeolites [11]. A specific mechanism of formation, where amorphous particles are first formed and then converted into crystals without changing the size, was observed [11b]. The formation of zeolite nuclei was detected in the centre of each particle and then the crystalline network propagated outward until complete transformation of the amorphous particles into crystalline particles had occurred. According to the X-ray diffraction (XRD) and transmission electron microscopy (TEM) studies these particles were fully crystalline. Only after heating at an elevated temperature larger cubic zeolite A crystals were obtained. A lot of research has been devoted to zeolite formation by this mechanism in organic template free systems [12].
An important breakthrough, based on the above-described mechanism, was reported very recently. Ultra small EMT-type zeolites were synthesized from an organic template-free Na-rich initial system [13]. The obtained plate-like hexagonal EMT-type nanosized crystallites exhibit a size of 15 × 4 nm along the a and c directions, respectively. These dimensions correspond approximately to 7 × 2 unit cells. This is the first synthesis in the colloidal precursor suspension in the presence of large amounts of Na. It is important to note that this spectacular result was achieved by fine control of the polymerization process during the preparation of the initial suspension. Furthermore, the hydrothermal treatment at a moderate temperature allowed the conversion of the amorphous precursor into crystalline EMT-type zeolites without favouring Ostwald ripening. The low temperature conditions were important not only to limit the growth of the crystals, but also to avoid the formation of competing phases such as LTA-, SOD-, GIS- or FAU-type [14]. It is disappointing to know that the EMT-type zeolite was obtained only by employing 18-crown-6 ether as a structure directing agent (SDA) [15], which is the major obstacle to the industrial use of the EMT-type zeolite.
The most industrially important zeolite, FAU-type, has also been successfully synthesized using this method [16]. Again the controlled polymerization of precursor species allowed the stabilization of the clear suspension of discrete gel particles, which were hydrothermally converted into ultra small (10–15 nm) zeolite Y and zeolite X crystals. Products with high crystallinity and micropore volume equal to micron-sized crystals were obtained. The possible applications of nanosized FAU-type materials are substantial due to the fact that they can be synthesized with high yield in the absence of organic templates.
The synthesis of nanosized zeolites under the conditions described above and with yields similar to their micron-sized counterparts obtained from hydrogel systems makes them suitable for industrial use. At present, the most important zeolitic materials are synthesized in the form of nanosized crystals. Fig. 1 depicts different sub-families of microporous materials that are synthesized in the nanosized form.

Zeolites and zeotype materials synthesized with nanosized dimensions. Note: TS-1 is a titanosilicate with a MFI-type framework topology.
3 Properties of nanozeolites: advantages and disadvantages
Nanosized zeolites are crystalline microporous solids with physicochemical characteristics similar to those of micron-sized crystals. In most cases, the decrease of crystal size is advantageous and extends the possible application of the zeolites. Fig. 2 summarizes the advantageous effects of nanosized dimensions of microporous crystals. However, the substantial decrease of zeolite crystal sizes could also have some negative consequences. For instance, crystallization under very mild synthesis conditions and the sole use of organic structure directing agents influence the framework composition. Zeolite materials synthesized under such conditions often exhibit a larger number of framework defects. Therefore, the preparation of nanosized zeolites under conventional conditions is of paramount importance for some practical applications.
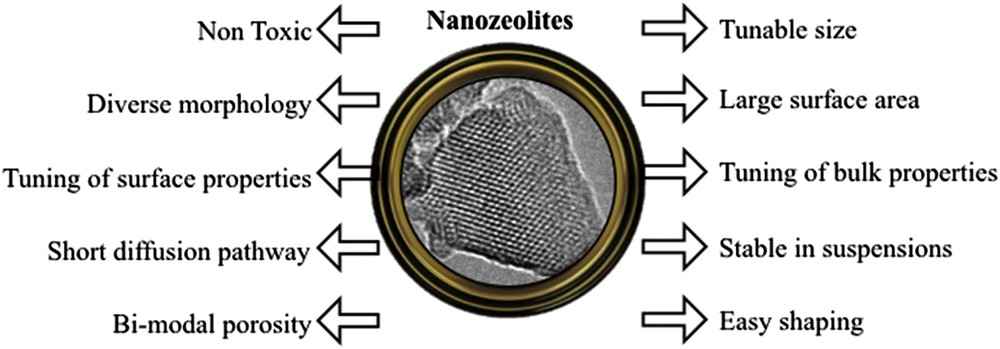
Properties of nanosized zeolites.
The substantial decrease of crystalline domains often results in broadening of the X-ray diffraction peaks, which is more pronounced in the case of particles smaller than 20 nm. Even very small nanocrystals exhibit a micropore volume similar to micron-sized ones. However, they display a much larger external surface area that could bring some benefits to the system but could also be disadvantageous. Large external surface areas are advantageous when molecules that are too bulky to pass through the pore window have to be processed. On the other hand, a large external surface could imply reactions that are not stereo-selective and thus may decrease zeolite selectivity. The short diffusion path through zeolite channels could also have both desired and undesired consequences. This improves the kinetics of the reaction and limits coke formation, but on the other hand zeolite selectivity is deteriorated.
Nanosized zeolites differ from micron-sized crystals in regard to post-synthesis treatment, which is required to avoid aggregation between the particles. The non-converted reactants are usually removed by high-speed centrifugation and redispersion of zeolite nanoparticles in distilled water. The procedure is repeated several times and then the pH of the resultant suspension is adjusted to be between 7 and 9. At this pH value the negative charge of the zeolite crystals is high enough to ensure colloidal stability. The repulsive forces of negatively charged particles protect the particles from aggregation for months and even years. Freeze drying is used when a dry powder of zeolite nanocrystals is needed. This process limits the aggregation between the crystallites.
4 Shaping of nanosized zeolites
Due to the very small size and high stability of nanosized zeolites in different solvents, they can be further processed and used for preparation of different constructs. For sorption and catalysis applications, the nanosized zeolites are usually shaped with the help of a binder (silica or alumina) in different forms. The bound nanocrystals can be further employed in a variety of shapes and forms including granules, particles, pellets, extrudates and monoliths with great micro-meso-macro- porosity. The nanosized zeolites synthesized from template free precursor suspensions with high crystallinity can be directly mixed with flocculants with high molecular weights that exhibit unique attraction towards colloidal silicates. In this case the nanosized zeolites do not require high-speed centrifugation and post-synthesis treatment to be shaped, due to the almost complete transformation of the amorphous aluminosilicates into crystalline particles.
A large research effort has been dedicated to the use of zeolite nanocrystals to prepare thin-to-thick zeolite layers for optical devices and gas/liquid sensors. The common preparation techniques for such devices include: (1) screen-printing, (2) sol-gel techniques, (3) dip- and spin-coatings, and (4) direct growth with or without pre-seeding of the substrates. The factors to consider when selecting the production technique include the required film characteristics, purity, porosity, reliability, reproducibility and costs. Zeolite films are used for the preparation of optical quality films with a thickness in the range of 50–170 nm through spin-on or sol-gel deposition methods [17]. Very recently an ink-jet printing approach has been used for preparation of zeolite-doped photopolymers for irreversible water sensors [17c]. Sensor devices with different shapes and security coding were prepared using ultra small zeolite nanocrystals.
Fig. 3 represents different products based on zeolite nanocrystals, including suspensions, thin films, self-supported or binder containing bodies.
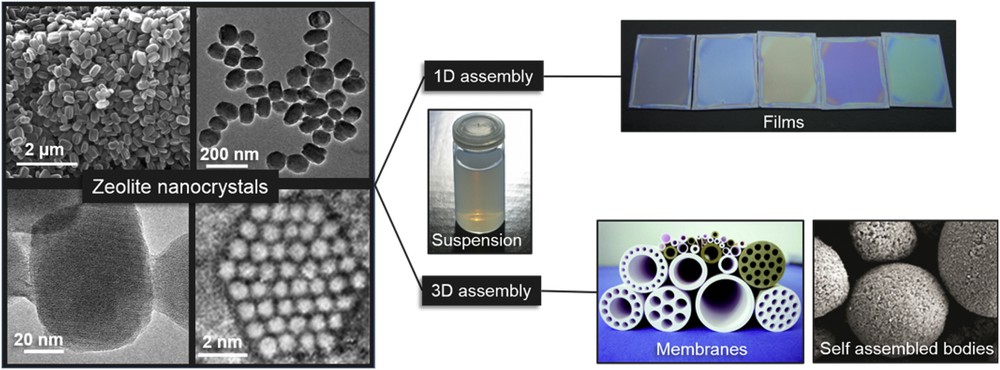
Nanosized zeolite crystals with a diverse morphology and size in colloidal suspensions, self-supported shapes, porous membranes, and optical quality films.
5 Advanced applications of nanosized zeolites
Recent developments in the synthesis procedures for zeolite nanosized crystals and their stabilization in suspensions, films, membranes, and hierarchical forms expand their applications to nanotechnology including sensors, optical layers, medicine, pharmaceutical industry, cosmetics and food.
5.1 Optical devices
The optical properties of the zeolite films, including refractive index, extinction coefficient, thickness, and hardness, are dependent mainly on the type of the zeolite nanocrystal and on the method of film preparation. The porosity of the zeolite films depends on the type of the crystalline structure, type of binder and preparation technique.
The fabrication of optical devices using zeolite nanoparticles has attracted considerable attention due to their rigid structure, high thermal stability and availability in different morphologies. Emphasis has been placed on the development of zeolite films as low-dielectric constant layers for (i) future generation computer chips, (ii) environmentally benign corrosion-resistant coatings for aerospace alloys, and (iii) hydrophilic and bacteriological coatings for gravity-independent water separation in space stations. Numerous applications of zeolites exhibiting either hydrophobic or hydrophilic properties with low or high ion exchange capacity have been discussed [17]. The zeolite layers show great mechanical stability and hardness, which make them interesting for membranes for seawater desalination and proton exchange membrane fuel cells.
Another use of nanosized zeolites is based on the encapsulation of organic or inorganic guest molecules in the channels of zeolite nanocrystals for light harvesting, and photovoltaic applications [18]. Photovoltaic solar cells based on quantum dots (CdS and PdS) encapsulated in the voids of FAU zeolite have been presented [19]. The activity of QDs-zeolite based devices was found to depend on the type of the QDs and their homogeneity within the zeolite matrix.
Another application for zeolite films as corrosion-resistant layers has also been reported [20].
5.2 Zeolite membranes
Zeolite membranes are used for gas–vapour and liquid–liquid separation. Both applications are important from industrial and environmental points of view. For instance, CO2 separation from different gas mixtures is the most significant environmental problem nowadays. Hydrogen is considered as a “green” alternative for hydrocarbon fuels. Consequently numerous studies have been carried out to extract hydrogen from H2/N2, H2/O2, H2/CH4 and multi-component gas mixtures.
Liquid–liquid separation on zeolite membranes is also a promising substitute for energy-intensive processes such as distillation. Different alcohol-water (methanol, ethanol, propanol, butanol, etc.) mixtures have been subjected to separation by zeolite membranes. Zeolite membranes discriminate molecular mixtures on the basis of molecular size or surface diffusion mechanisms. In particular, the mechanisms are described as (i) molecular sieving, (ii) diffusion-controlled and (iii) adsorption-controlled permeation. Diffusion- and adsorption-controlled permeation govern the separation mechanism when the size of the pores is larger than the molecules to be separated. Additionally, sodalite–polyimide membranes with good hydrogen permeability and selectivity over nitrogen at the same testing temperature have been prepared [21].
Zeolite crystals with controlled orientation exhibit new properties, since their porous framework is capable of orienting and restricting rotation of the adsorbed guest molecules [22,23]. A significant improvement in the separation of the p-/o-xylene mixture in the c-oriented MFI membrane compared with the conventionally calcined membranes has been reported. Also, the oriented zeolite membrane prepared via secondary growth gave an excellent cumulative O2 permeance. A number of comprehensive reviews dedicated to the preparation and characterization of zeolite-based membranes have been published [23].
The great expectations for zeolites as separation media are based on their selectivity, long-term stability at high temperature, resistance to harsh environments or to high pressure drop, inertness to microbiological degradation, and catalytic activation.
The preparation of zeolite membranes, in particular, on a large scale, remains challenging. The major disadvantages of zeolite membranes are poor reproducibility, very often poor fluxes and technical difficulties related to sealing at high temperature. The substantially higher costs of zeolite membranes are also an obstacle to their large industrial utilization. Obviously, the processing methods will have to be further developed in order to ensure an industrial application of supported zeolite membranes [24].
Despite this, defect free cylindrical zeolite membranes are already commercially available (Mitsubishi Chemical) and they are used to remove impurities and to separate water from organic chemicals.
5.3 Zeolite-based sensors
Extensive research has been devoted to the selective chemical sensing of exhaust gases, hydrocarbons and bio-molecules using zeolite nanocrystals assembled in films. The current state-of-the-art in using porous materials and in particular zeolites for sensing applications was presented in a recent review [25]. Numerous examples for the use of zeolites in the sensing of water, oxygen, NOx, CO (CO2), hydrocarbons, and volatile organic compounds, were presented. The variation of pore dimensions, chemical composition, and crystal size of zeolites enabling the detection of different gases is ascribed to the size and shape selectivity of the materials.
The zeolites used for the preparation of selective chemical sensors are presented in Fig. 4. The detection of VOCs and hydrocarbons by zeolites under different conditions have been reported [26].

Selective chemical sensors for (left) bio-molecules and exhaust gases based on nanosized zeolites with (right) the corresponding principal of detection.
Size and shape selectivity towards different analytes is generally improved through control of the dimensions and shapes of the pores enabling detection and discrimination between different molecules. The chemical selectivity and sensitivity of zeolite-based sensors can also be controlled by changing the chemical composition of nanosized zeolites from pure silica to highly-aluminium containing zeolites, thus going from highly hydrophilic to hydrophobic materials. The incorporation of selected dopants such as metals, metal oxides, metal sulphides and organics into the zeolite frameworks can further improve the selectivity of the materials. Indeed, the defined zeolite pore structure makes them attractive as true shape-selective molecular sieves. The effect of acidity and pore diameter of zeolites on the detection of base molecules including water, acetonitrile, ammonia, benzonitrile, pyridine, aniline, and triethylamine has been investigated [27]. It was pointed out that acid–base interaction is the major factor when the molecular diameter is smaller than the zeolite pore diameter. As for larger molecules (benzonitrile, pyridine, aniline, and triethylamine) for which the molecular diameter is nearly the pore diameter of zeolites, the response magnitudes were lower than those of the smaller molecules. The sensitivity of the zeolite sensors to various base molecules as a function of proton affinity, which can be regarded as a measure of base strength of the molecules, has been investigated. The sensitivity generally increased with the increase in the proton affinity. In the case of large molecules such as pyridine and triethylamine, the sensitivity was strongly dependent on the type of zeolites (MOR > BEA > MFI) indicating the significant contribution of diffusion in the detection of larger molecules. Additional functionality in the zeolite sensors has been introduced by using their ion-exchange properties and thus the selectivity towards desired molecules was achieved [28].
The sensing devices have to be electrically conductive, catalytically selective, and electrochemically active. Moreover, the devices have to exhibit high long-term stability under harsh environmental conditions. Zeolite-based sensors for exhaust gases including nitrogen oxides (NOx), carbon monoxide (CO) and carbon dioxide (CO2), hydrocarbons such as methanol, ethanol, aldehydes and alkanes, ammonia (NH3), hydrogen sulphide (H2S), sulphur dioxide (SO2) and hydrogen (H2) have been reported in several studies and review articles [29].
5.4 Biological and medical applications of nanosized zeolites
Prior to using zeolite nanocrystals for medical applications, the anticipated question is whether these materials are safe since their size is considerably reduced (10–100 nm). The cytotoxicity of inorganic nanoparticles including zeolites, metals and metal oxides is recognized as an important issue in “nanosociety”. Nanoparticles in general may have an adverse effect on human health compared to their micrometer counterparts and therefore nano-safety regulations become necessary. Nanoparticles can enter the human body via the lungs where a rapid translocation through the blood stream to vital organs is possible, including crossing the blood brain barrier (BBB), and by absorption in the intestinal tract or through the skin.
Most of the toxicity tests have been performed on five types of zeolites (LTA, MOR, LTL, FAU and MFI). It was shown that these zeolite nanocrystals have low cytotoxic activity, although they have different sizes, compositions and shapes [30]. These results are expected to further expand the future industrial and medical applications of nanosized zeolites. The nanosized zeolites (LTL and EMT) free of organic templates show no toxicity to HeLa cells [30,31]. Pure silica zeolites and low-Al containing zeolites were reported to be non-toxic no matter how much was used. Most of the measurements were carried out with a HeLa cancerous cell line.
The biocompatibility of the nanoparticles was also investigated by analysing their effect on the biological properties of cellular proliferation, cytotoxicity and both extracellular and intracellular free radical generation capacity [32]. DNA fragmentation analysis disclosed that the MFI nanosized zeolites cause genotoxicity in a concentration dependent manner. This study therefore pointed towards the capability of the non-cytotoxic zeolite nanoparticles to induce oxidative stress resulting in short-term altered cellular metabolism up-regulation and genomic instability. The conclusion drawn from this study was that the pure silica MFI-type zeolites were relatively non-reactive and cytotoxic to the human alveolar cells.
It is expected that non-toxic zeolite nanocrystals with interesting physicochemical properties may offer considerable benefits in everyday life uses. The anti-bacterial activity of silver loaded FAU-type zeolites against Escherichia coli (E. coli) was reported, thus showing an increase in bacterial susceptibility in the case of rifampicin [33]. The stabilisation of Ag within the zeolite matrix resulted in enhanced antifungal activity in comparison with free Ag+ ions. Additionally, patterned-zeolite films consisting of FAU zeolite crystals were also found to be efficient towards eradication of E. coli. Another example is a nitrogen oxide (NO) loaded Zn-containing zeolite, which was also used as a bi-functional anti-bacterial material [34].
The immobilization of bio-molecules on the surface of zeolite nanocrystals is a focus of intense activity in biotechnology and biomedicine [35]. Zeolite nanocrystals also show great promise as substrates to immobilize proteins due to their small size and high functional group density for further grafting. The hydrophilic/hydrophobic nature, high stability in colloidal suspensions, and high adsorption capacity of zeolite nanocrystals make them important for further applications in the pharmaceutical industry and medicine. Drugs or contrasting agents can be confined within the pores or cages of zeolites. There are cases where the drug of interest is attached to the external surfaces, and therefore the interest is in the synthesis of zeolites with high external surface areas. The application of zeolite nanocrystals for biomedical applications, controlled carry and delivery of probes are envisioned for theranostic and gene therapy applications too [35]. Potential biochemical and medical applications of nanosized zeolites are summarized in Fig. 5.

Biochemical and medical applications of nanosized zeolites.
New horizons in clinical medicine have been opened using nanosized zeolites, in particular the multifunctional therapeutic delivery systems. The ability to store and release a variety of drugs in the FAU-type zeolites was reported [36]. The FAU-type zeolite demonstrated slow release for various anthelmintic drugs. The controlled release of sulfonamide antibiotics adsorbed on the zeolite crystals was investigated by changing the pore mouth opening, modifying the external surface, and the nature of the grafted functional groups [36].
Immobilizations of different molecules in zeolite structures suggests that the kinetics can be controlled by varying the incubation time, drug concentration and pH of the solution [37]. Vectorized therapies for tumours based on inorganic nanomaterials have recently been proposed to be an exciting approach. Nanoparticles with vectorization properties have been used to deliver different drugs to tumours. This suggests that amongst the newly developed nanomaterials, zeolite nanocrystals will be particularly interesting for vectorization.
Other applications for nanozeolites include storage and controlled delivery of gases in biological systems [38]. Reversible adsorption is a good method for storing a desired gas in a material from which a controlled delivery is required. Zeolite crystals have been used to trap nitrogen from compressed room air, and thus concentrate the oxygen in the expressed gas. At delivery flow rates of 2–5 l per minute, oxygen concentrations greater than 80% were maintained. The possibility of using zeolites for oxygen concentrators was demonstrated with a packed LTA zeolite, which produces humidified O2-enriched air because H2O is adsorbed and recovered together with the oxygen. The tuneable nature of NO release from ion exchanged LTA zeolites extends their potential applications to tubing and instruments used in procedures such as bypass surgery and renal replacement therapy [38].
An effort to enhance oxygen delivery via using fluorinated zeolite particles embedded in three-dimensional polyurethane scaffolds was exploited [39]. It was shown that polyurethane scaffolds with zeolites could be used for tissue engineering with enhanced oxygen delivery to the cells. Artificial mechanical red blood cells mimic the oxygen and the carbon dioxide function as transport of red blood cells. They can deliver more than 200 times as much oxygen per unit volume than a natural red blood cell.
A very recent work demonstrated the affinity of zeolite nanoparticles possessing a high surface area and high pore volume towards human plasma proteins [40]. The protein composition of the zeolite nanoparticles was shown to be more dependent on the plasma protein concentration and the type of zeolite than the zeolite concentration. The number of proteins present on the surface of zeolite nanoparticles at 100% plasma is less than with 10% plasma exposure. This was explained by the competition between the proteins to occupy the surface of the zeolite nanoparticles. Moreover, a high selective adsorption for apolipoprotein C-III (APOC-III) and fibrinogen on zeolite nanoparticles at high plasma concentration (100%) was observed. The zeolite nanoparticles exposed to low plasma concentration (10%) exhibited a highly selective adsorption for immunoglobulin gamma (i.e. IGHG1, IGHG2 and IGHG4) proteins. It was demonstrated that the zeolite nanoparticles can be used as additives to hemophilic patients (hemophilia A (F-VIII deficient) and hemophilia B (F-IX deficient)) with a risk of bleeding, and thus they might potentially be used in combination with existing therapies.
6 Conclusions and perspectives
In conclusion, nanosized zeolites are poised to play an important role in the well-known classical applications of catalysis, separation and ion exchange processes. New fields of application are also emerging because of the exceptional properties of nanozeolites and their potential to be supplied in various forms, such as suspensions, optical quality thin films, membranes and self-supported morphologies.
The new synthesis strategies for nanosized zeolites have been rationalized and they will be used to further extend the number of zeolites with nanosized dimensions. Substantial progress has been made in the processing of nanosized zeolite from template free precursor suspensions resulting in highly crystalline materials in high yields comparable to those of commercial micron-sized zeolites. Circumventing the use of an organic template is an important step ahead in the future extensive application of nanosized zeolites. Post-synthesis steps will be further introduced in the nanosized zeolites in order to tailor their final properties. A large commercial scale for some of the nanosized zeolites, especially once free of organic templates, is envisaged.
At present the available nanozeolites (20 types) offer a tuneable crystal size and chemical composition, diverse morphology, large external surface area, fast diffusion, high stability in suspensions, and no toxicity, which will result in an increase in their applications. The applications of nanosized zeolites in chemical sensing, biomedical and biological analyses have already shown great potential. Their unique surface chemistry, high thermal stability, high surface area and large micropore volume are important for the design of optical devices. Useful strategies for functionalization of zeolite nanoparticles open up the possibility of using the materials in areas such as environmental drug delivery, medical imaging and other biomedical applications. The low cytotoxic activity of nanosized zeolites with different sizes, compositions and shapes has been reported and this is expected to enlarge their future industrial and medical applications. The engineering of devices and systems based on zeolite nanocrystals will continue to involve ever–greater interactions and collaborations between chemists, physicists, materials scientists and engineers.
The main tendencies and challenges in the preparation and application of nanosized zeolites are presented in Fig. 6.

Nanosized zeolites: main trends and challenges related to their processing, characterization and applications.
In conclusion, the desire for new porous materials and more specifically zeolites with original properties will continue. Millions of hypothetical zeolite structures have been forecast and this will further motivate synthetic chemists to develop different approaches for their preparation based on a profound understanding of the zeolite crystallization mechanism.
Acknowledgments
The financial support from the Lower-Normandy Region, TARGED ANR-13-BS08-0002-01 and MicroGreen ANR-12-IS08-0001-01, is acknowledged.