1 Introduction
In the last decade, much attention has been paid to the eradication of various environmental pollutants such as dyes, pesticides, detergents, and volatile organic compounds from wastewater [1–4]. Traditional physical techniques such as adsorption on activated carbon, reverse osmosis, coagulation by chemical agents, and ion exchange on synthetic adsorbent resins, have been used for the removal of pollutants [5,6]. However, these methods only succeed in transferring organic pollutants from water to the solid phase. Further, secondary pollution is created, which entails additional and costly solid-waste treatment and regeneration of the adsorbent. Therefore, an alternative to conventional methods is needed. In this regard, photocatalysis, as an advanced oxidation process, is one of the promising alternative methods applied in this field.
It is based on the photogeneration of reactive species such as hydroxyl radicals (•OH) which can oxidize a broad range of organic pollutants quickly and non-selectively [7]. Due to its high chemical stability, non-toxicity, high photocatalytic activity to oxidize pollutants in air and water, and relatively low price, TiO2 has been widely used as a photocatalyst in recent decades [8–11]. However, its application is limited because of its narrow photocatalytic region (near-ultraviolet region) and small absorption fraction (<5%) of incident solar irradiation and indoor light [12–14].
Moreover, the extreme low coverage of organic pollutants on the TiO2 surface is another limitation of the low photocatalytic efficiency [15,16].
To improve the affinity between organic pollutants and the TiO2 surface, aromatic carboxylic acids such benzoic (BA) and salicylic (SA) acids are usually used as surface modifiers. Particularly, the TiO2 surface modification by SA may enhance the surface coverage of organic pollutants, expand the wavelength response range and improve the dispersive capacity of TiO2 powder in polar and non-polar solvents [17].
Carboxylic acid-modified TiO2 photocatalysts are always bulk particles or powder materials [18–21]. They always sink or suspend into the solution leading to a reduction in the fraction of sunlight used in the photocatalytic reaction. Furthermore, a common drawback of suspended catalysts is the difficulty associated with the recycling, which usually limits their practical applications. In this paper, a new concept is developed called the “floating photocatalyst” to solve the above limitations. The designed floating photocatalyst consists of surface-modified TiO2 immobilized on a floatable substrate (Scheme 1). The floatable photocatalysts are especially interesting for solar remediation of non-stirred and non-oxygenated reservoirs since the process maximizes: (i) the illumination/light use (due their ability to float), and (ii) the oxygenation of the photocatalyst due to the proximity to the air/water interface. The optimization of illumination and oxygenation should result in higher rates of radical formation and oxidation efficiency. Floating photocatalysts can be applied for local solar remediation, i.e. directly in the contaminated wastewater reservoirs located in remote areas without any special equipment or installation. Also, they can be used for more efficient destruction of suspended insoluble organic contaminants, e.g., in oil-spill accidents [22]. Palm trunk PT is a natural material of low density, antistatic properties and heat insulation. So, it can be used efficiently as a floating substrate. PT is a suitable support for photocatalytic applications since it is an innocuous material with good chemical, mechanical and thermal stability. Moreover, PT is a hydrophobic material and can pre-concentrate organics from water on its surface improving the removal and oxidation efficiency. PT with a highly developed porous structure formed by the inter-lamellar space has been used for different applications as an adsorbent and absorbent.
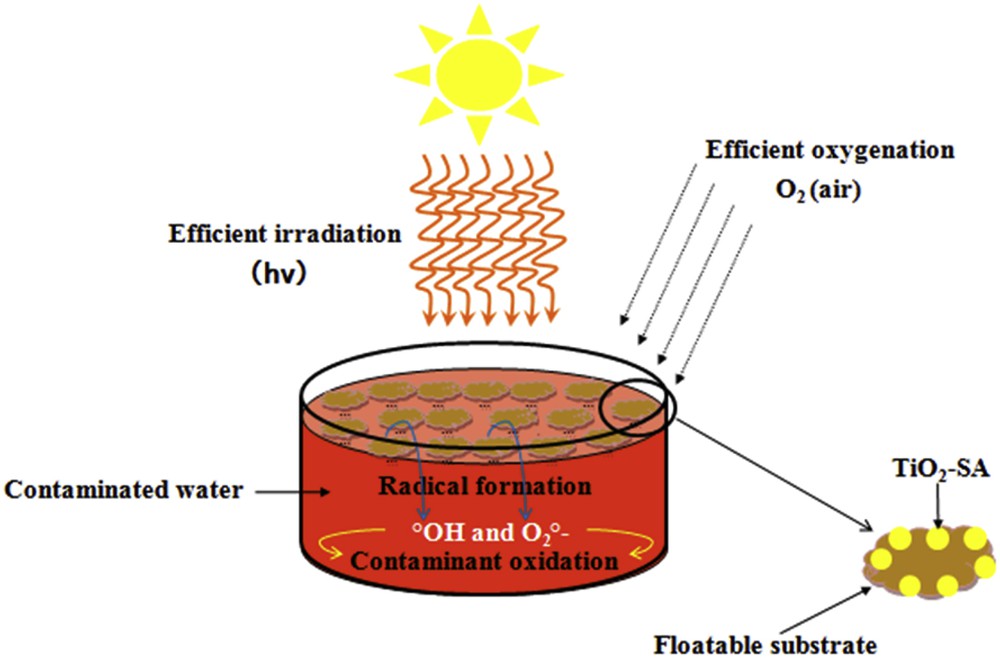
Schematic representation of the floating photocatalyst used for the oxidation of CR dye.
The aim of this research work is then to prepare a TiO2–SA/PT as a floating photocatalyst and to examine its efficiency in photodegradation of pollutants under solar light and constrained conditions (non-stirring and no artificial oxygenation).
2 Materials and methods
2.1 Materials
All reagents used were of analytical grade and employed as received. Titanium tetraisopropoxide (TTiP) and sodium dodecyl sulfate (SDS) were purchased from Aldrich Chemicals. Ethanol, salicylic acid (SA) and acetic acid were obtained from Merck Chemicals. The azo dye used in the experiments is Congo red (CR). PT was obtained from palm oases of Gabes (Tunisia).
2.2 Preparation of the TiO2 photocatalyst
The TiO2 photocatalyst was prepared through the sol–gel process. The two precursor solutions, here denoted as precursors A and B were prepared as follows. Precursor A: TTiP (22.5 mL), ethanol (50 mL) and acetic acid (3.0 mL). Precursor B: H2O (27.0 mL), ethanol (50 mL) and SDS (0.43 g). Each precursor was stirred for 10 min at room temperature. Afterwards, precursor B was added to precursor A dropwise with vigorous stirring. The resultant mixture was further stirred for 20 min. The sol solution was placed in a culture dish for 1.0 day to finish the sol–gel transition and filtered. The filter residue was rinsed with water repeatedly, and then dried at 110 °C for 24 h to obtain a dried gel. The dried gel was ground and heat treated at 350 °C for 6.0 h to get a stable TiO2 photocatalyst.
2.3 Surface modification
Surface modification was carried out through adding 1.0 g of the TiO2 nanoparticles into 50 mL of saturated solution of SA. A constant magnetic stirring was conducted for 30 min at room temperature until the adsorption equilibrium, resulting in a light yellow coloration of the surface of TiO2, inferring that a chemical reaction took place (chemisorption) between SA and TiO2. After being filtered, the surface-modified titania nanoparticles (TiO2–SA) were washed with water three times and heat treated for 30 min at 105 °C. The obtained powder was then ground to get fine particles.
2.4 TiO2–SA/PT photocatalyst preparation
The floating photocatalysts TiO2–SA/PT were prepared from a suspension of TiO2–SA on the PT surface. The PT was divided into small pieces. Different TiO2–SA contents of 50, 70 and 100 wt % were used. The immobilization of the photocatalysts on PT pieces was performed under vigorous stirring at room temperature; by dissolving 3.0 mL of binder in 30 mL distilled water containing 1 g of PT. Then, an appropriate amount of TiO2–SA was added. The resultant mixture was further stirred vigorously for 30 min. After filtration, the recovered product is dried in an oven at 60 °C for 5.0 h. The TiO2–SA/PT beads were subsequently subjected to vigorous stirring in water for 2.0 h to remove any TiO2–SA particle loosely attached to the PT surface.
2.5 Catalyst characterization
The TiO2 nanoparticles and TiO2–SA nanocomposites were characterized by X-ray diffraction (XRD), scanning electron microscopy (SEM), thermogravimetric analysis (TGA), Fourier transform infrared spectroscopy (FTIR), and diffuse reflectance spectroscopy (DRS). The XRD patterns obtained on a D5000X-ray diffractometer (German Bruker) using Cu Kα radiation at a scan rate of 0.02° s−1 were used to decide the crystallite size and identity of TiO2 powders. The average crystallite size was determined according to Scherrer's equation:
2.6 Photocatalytic experiments
A special photocatalytic reactor of 250 mL with an outside diameter (∅out = 90 mm), height (H = 45 mm) and covered walls was designed to produce only surface illumination avoiding lateral light incidence. The reactions were carried out under natural sunlight irradiation (out in the city of Gabes, in the month of July between 10 am and 2 pm). The photocatalytic studies were carried out with the reactive textile dye CR as a probe molecule at 10 mg L−1 concentration. Before the reaction, the catalyst was kept in the dye solution (100 mL, 10 mg L−1) in the dark for 60 min to reach adsorption equilibrium. No stirring was used in the reactions in order to avoid oxygenation of the solution and to simulate the use of a floating photocatalyst. During the photodegradation process, the samples were collected every 30 min, and the catalyst was removed by centrifugation. An UV–Vis spectrometer (UV-2550, Shimadzu, Japan) was used to measure the organic dye concentration before and after degradation. The degradation efficiency (D) was calculated using the following equation [23,24].
3 Results and discussion
3.1 XRD analysis
XRD analysis of the prepared pure TiO2 and modified TiO2–SA nanocomposites (Fig. 1) was conducted to investigate the influence of SA deposition on the crystalline phase of TiO2 nanoparticles. Fig. 1a reveals a unique anatase solid phase characterized by the principal peaks observed at 2θ = 25.3, 37.9, 48.1, 54, 55.2 and 62.8° which exactly match (101), (004), (200), (105), (211) and (204) reflections of the pure anatase phase. The characteristic peaks of rutile and brookite phases are not observed, revealing that these phases do not exist in the as-prepared TiO2. Fig. 1b shows that the modification of the TiO2 particles by SA does not cause any change in their peak positions and shapes compared to TiO2. This indicates that SA modification through impregnation at room temperature does not change the crystalline structure of TiO2. The average crystallite size of TiO2 and TiO2–SA particles, calculated using Scherrer's equation is found to be equal to 5.22 nm and 5.16 nm, respectively. Broad diffraction peaks are due to the small crystalline size of TiO2–SA particles.
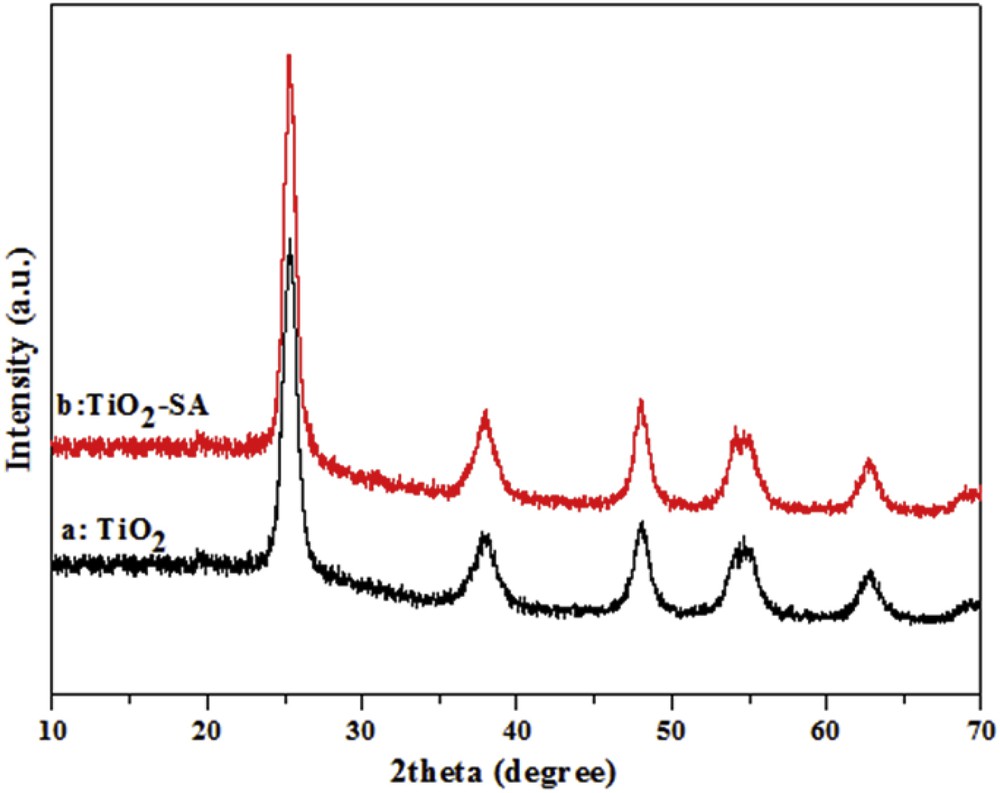
XRD patterns of the prepared pure TiO2 (a) and modified TiO2–SA nanocomposites (b).
3.2 SEM images
SEM images were recorded for unmodified TiO2 and TiO2–SA nanocomposites to investigate the effect of surface modification on the morphology and aggregation level of nanoparticles (Fig. 2). The SEM images show that TiO2 particles are more aggregated than the modified TiO2–SA ones. The average size of TiO2 aggregates is 1 μm (Fig. 2a). The synthesized TiO2–SA nanoparticles appear less aggregated, small in size and have a spherical-like shape with a uniform size distribution (Fig. 2b). A higher dispersion increases the specific surface area, which ultimately enhances the photocatalytic performance on the TiO2–SA nanopowders.
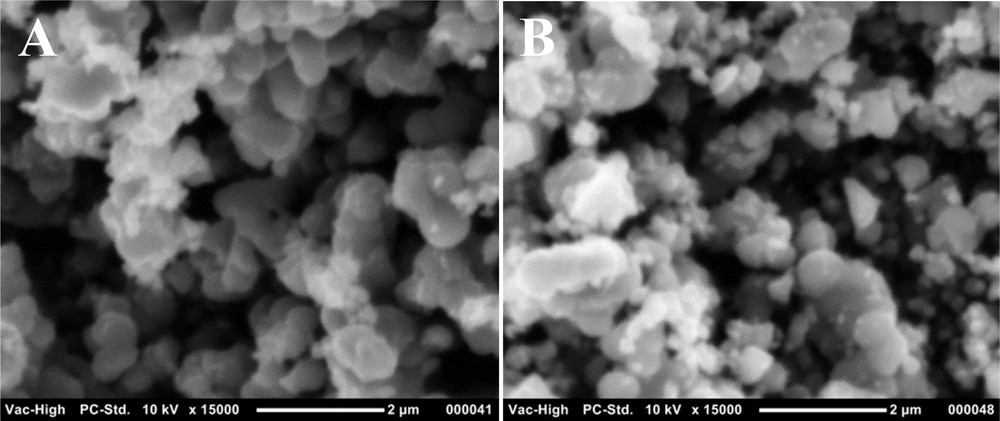
SEM images of (A) prepared pure TiO2 and (B) modified TiO2–SA nanocomposites.
3.3 Thermal stability
The amount of SA adsorbed on the TiO2 surface was deduced from thermogravimetric analysis of prepared pure TiO2 and TiO2–SA nanocomposites (Fig. 3). The TGA curve of the prepared pure TiO2 (Fig. 3a) shows thermal stability up to 500 °C where the loss of mass is negligible (1%) and may due to some humidity. A larger mass loss of about 4% is registered with the TiO2–SA nanocomposite (Fig. 3(b)) and corresponds to the decomposition of the adsorbed SA. As expected, the nanocomposite TiO2–SA has become more resistant to thermal degradation, probably because of the strong interactions between the TiO2 and SA.
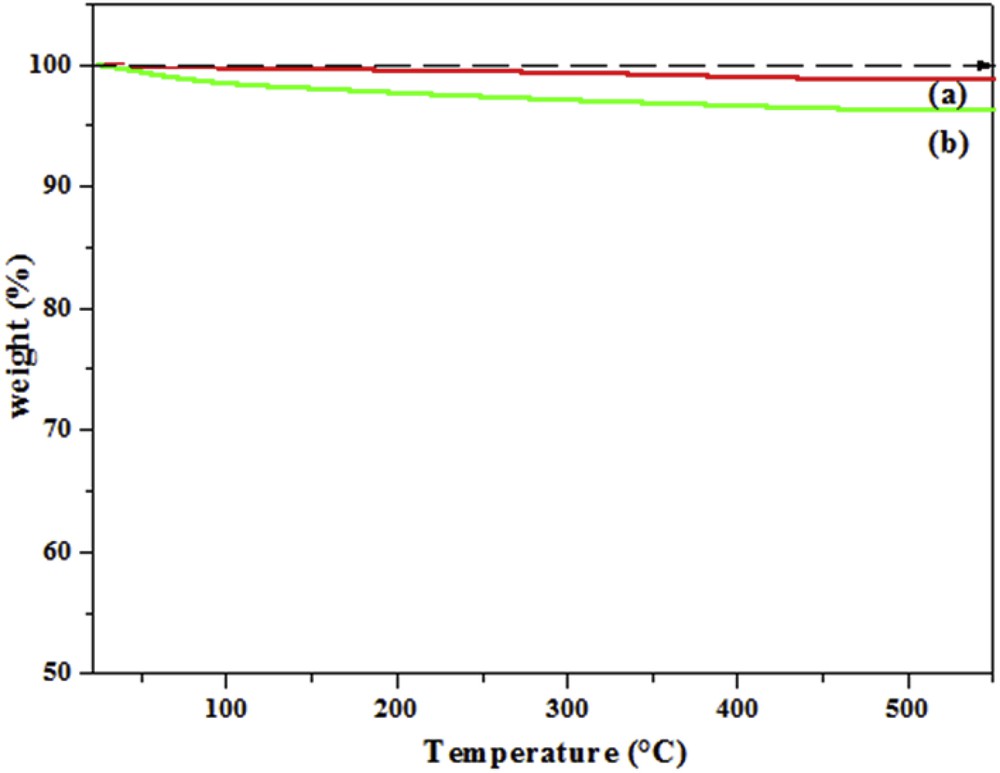
TGA curves of (a) prepared pure TiO2 and (b) modified TiO2–SA nanocomposites.
3.4 FTIR analysis
Fig. 4 depicts the FTIR spectra of the prepared TiO2 powders before (a) and after (b) modification. The FTIR spectrum of pure TiO2 shows the deformation frequencies of OH functions of adsorbed water located at 1674 and 3462 cm−1. The peak located at 1111 cm−1 is attributed to the stretching vibration of the Ti−OH bond whereas the absorption peak at 576 cm−1 is a characteristic of the Ti−O bond vibration [25]. The spectrum of TiO2 modified by salicylic acid (TiO2–SA) shows new peaks allocated to CO of the ester group (1711 cm−1), phenyl (1441 and 1474 cm−1) group and −COOTi− group (1385 cm−1). This result suggests the formation of a fairly stable complex between TiIV surface ions and salicylic acid. The formation of this complex is also shown by the appearance of a yellow coloration on TiO2 particles that can be explained as a result of a ligand to metal charge transfer transition. Indeed, salicylic acid shows a great affinity to TiIV ions in solution and chemisorbs efficiently at the titanium dioxide surface. The combination of a −OH group and a −COOH group at ortho positions of the benzene ring infers some strong interaction between the salicylic acid and the TiO2 particle surface. The dissociation constants of salicylic acid are pKa1 = 2.81 and pKa2 = 13.4. As a result, the −COOH group of salicylic acid is dissociated into −COO− and H+. The TiIV surface ions of the TiO2 typically bond with the oxygen atoms of water molecules. However, in the presence of salicylate anions in solution, the −COOH and the −OH groups form chelate surface structures with the surface TiIV ions leading to the replacement of water by the organic ligand [26]. Two structures of the surface complexes are proposed: bidentate chelating and less stable bidentate bridging, or monodentate through the oxygen atom of the carboxyl group [27–29]. A chelating bidentate surface salicylate species, coordinated via a C–O bond of the carboxylate group, is the most likely resulting structure at the TiO2 surface [30]. Shun-Xing [17] established that salicylic acid and −OH groups on the TiO2 surface interact through the esterification reaction leading to the formation of ester with a stable six-membered ring. The formation of such a surface complex TiO2–SA can lead to a shift of absorption edge toward the visible spectrum up to 430 nm.
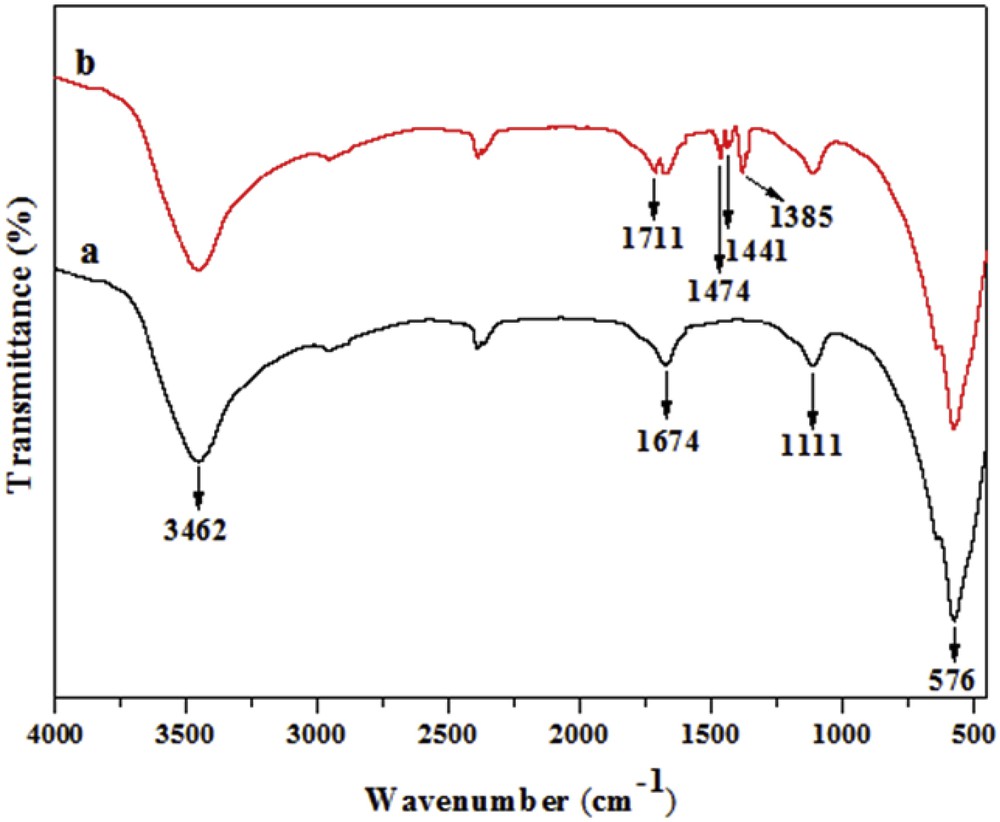
FTIR spectra of (a) prepared pure TiO2 and (b) modified TiO2–SA nanocomposite.
3.5 DRS analysis
Fig. 5 shows the DRS of the prepared pure TiO2 and modified TiO2–SA nanocomposite. It is obvious that pure TiO2 absorbs only light with wavelengths below 380 nm. However, the modified TiO2–SA nanocomposite exhibits strong absorption in the visible range (from 400 to 800 nm), which conforms to the light yellow color of the powder. Notably, the absorption edge of the TiO2–SA red-shifts about 40 nm compared to that of the neat TiO2 sample. The DRS result indicates that electron transfer between SA and TiO2 nanoparticles exists in TiO2–SA, which might be favorable for the improvement of the photocatalytic activity of TiO2. The band gap energies (Eg) of the pure TiO2 and modified TiO2–SA nanocomposite were 3.2 and 2.9 eV, respectively. The band gap energies of the photocatalysts were determined using the following equation: Eg (eV) = 1239.8/λ [31], where λ is the wavelength (nm). The above results show that SA is capable of sensitizing TiO2 efficiently. Consequently, the TiO2–SA nanocomposite can be excited to produce much more electron–hole pairs under visible light illumination, resulting in higher photocatalytic activities. This also shows that SA modification provides an effective approach to extend the absorption of TiO2 to the visible light range.
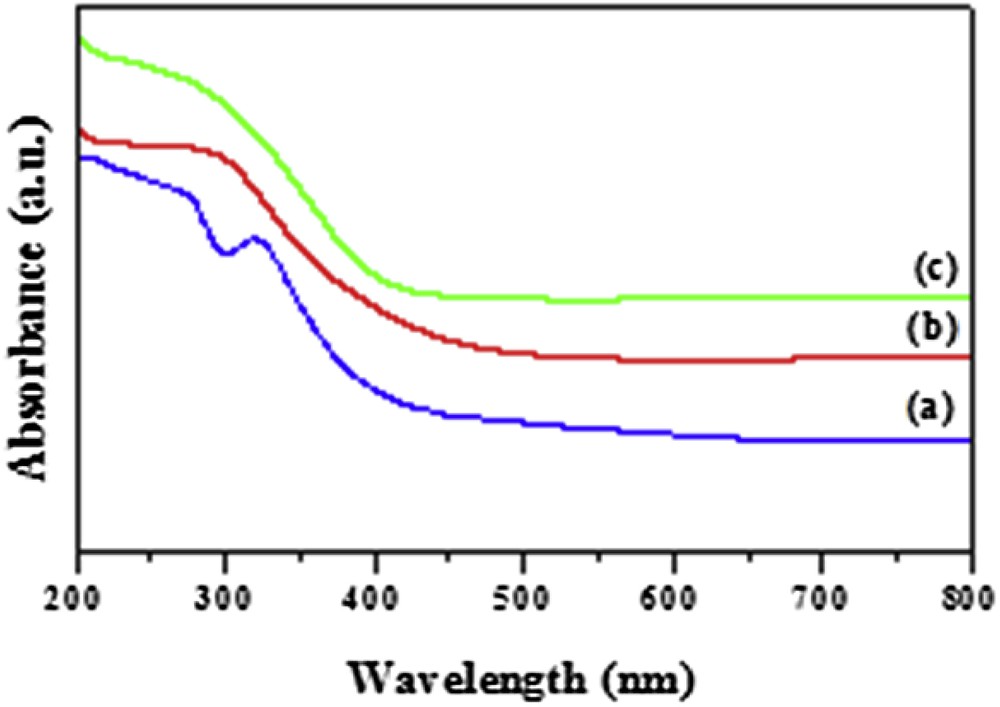
UV–Vis diffuse reflectance spectra of (a) pure SA; (b) prepared pure TiO2 and (c) modified TiO2–SA nanocomposite.
3.6 Effect of solution pH
The pH of the solution is a vital parameter in the photodegradation of organic dyes because it determines the surface charge of the photocatalyst, the electrostatic interaction between the photocatalyst surface and organic dye molecule, and the number of charged radicals generated during the photocatalytic oxidation process [32]. In addition, the initial solution pH may affect the aggregation of the particles and the conduction and valence band positions of the catalyst. To decide the effect of the pH value of organic dye solution on the photocatalytic performance, a series of comparative photodegradation of CR were performed in the presence of TiO2–SA/PT, TiO2/PT, TiO2–SA and pure TiO2 under solar light irradiation. The studies were carried out at a pH value ranging from 4 to 10. The pH of the solutions was adjusted with HCl or NaOH solution by using a pH meter.
Fig. 6 shows the degradation efficiency at different pH values. The maximum of the photocatalytic performance was acquired at pH = 6 for all the photocatalysts tested here. In particular, TiO2–SA (50 wt %)/PT shows the highest degradation efficiency (84.2%) of CR after 100 min of irradiation. This result can be explained taking into account the charge surface state of TiO2–SA and the ionic state of CR in the solution. CR molecules with two sulfonic groups ionize easily in strong acidic media and become a soluble CR anion, which can be easily adsorbed on TiO2–SA particles with positive surface charge. So, the maximum degradation ability of TiO2–SA/PT under solar light was measured under the optimum experimental conditions: concentration of the organic dye (CR) = 10 mg L−1 and pH = 6.
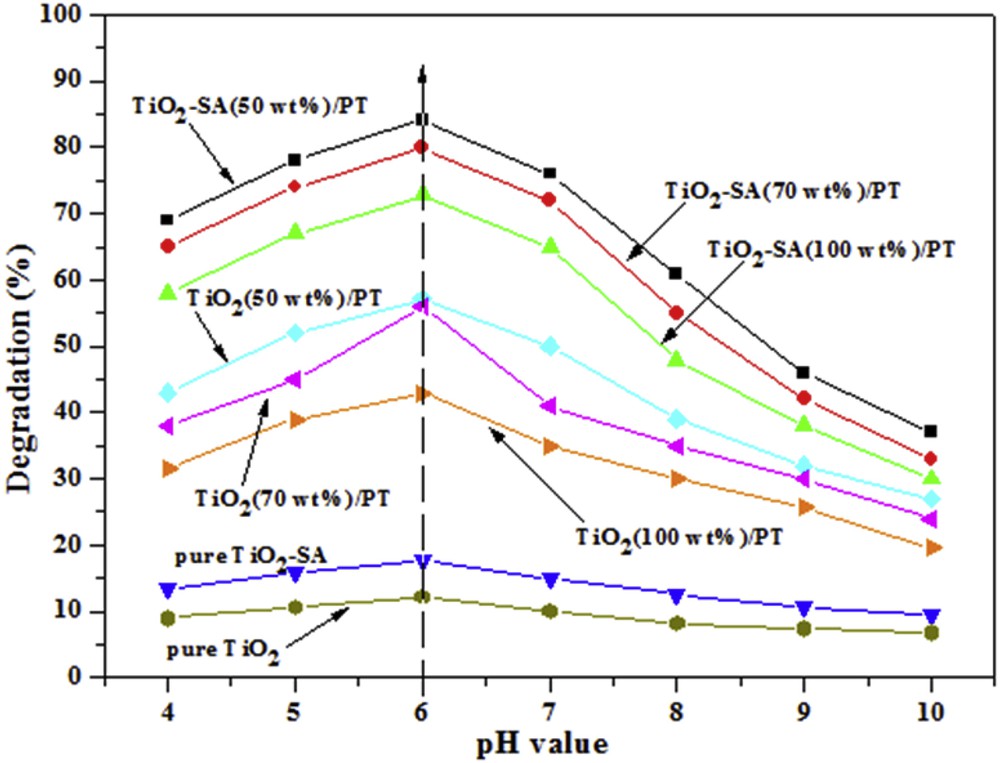
Effect of pH values on the photodegradation of CR after 100 min (Experiments were carried out under solar light irradiation, T = 34 ± 2 °C, Volume = 100 mL; initial concentration of CR = 10 mg L−1; catalyst dose = 1.0 g L−1).
3.7 Photodegradation of CR
Fig. 7 shows the concentration variation of CR solution when irradiated with solar light in the presence of different catalysts. Before photocatalytic tests, the dye solution was left in contact with the photocatalyst for 1.0 h in the dark to reach the adsorption equilibrium. The blank test (in the absence of catalyst) reveals that the decrease of the CR concentration due to its photosensitization property is not significant. Besides, Fig. 7 shows that the adsorption potential of SA- modified TiO2 is higher than that of the unmodified TiO2. According to the SEM and DRS studies, the TiO2–SA particles have a small size compared to pure TiO2, resulting in a higher surface area. Thus, TiO2–SA provides more sites for the adsorption and the removal of the organic molecules.
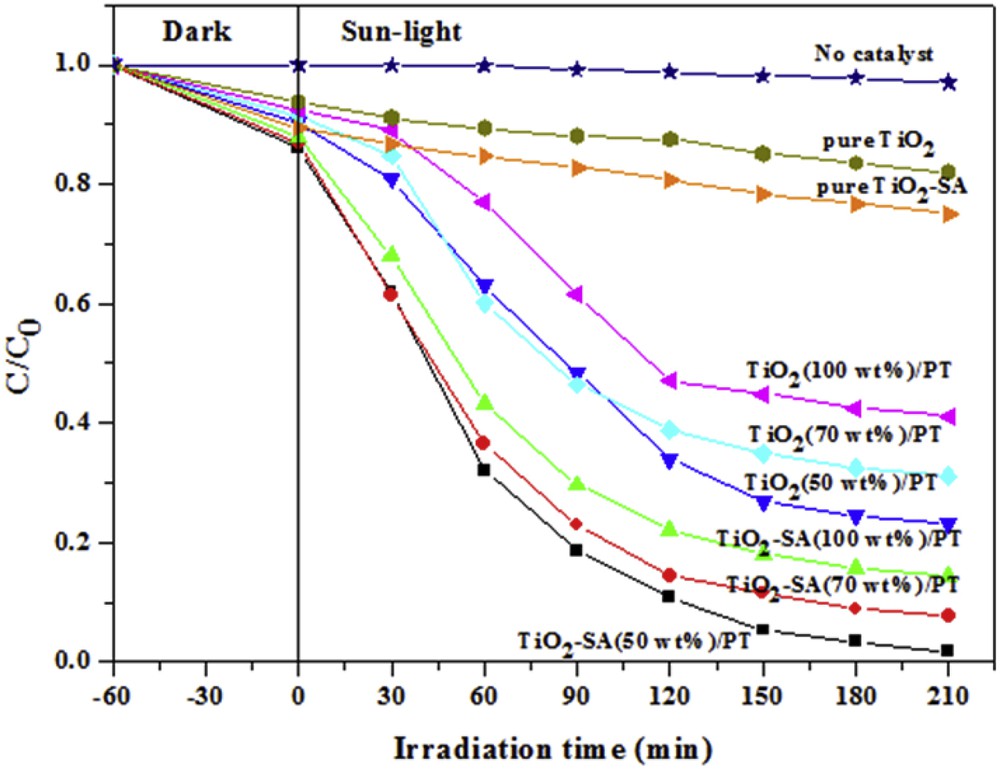
Photocatalytic degradation curves of CR in the presence of different catalysts. (Experiments were carried out under solar light irradiation, T = 34 ± 2 °C, volume = 100 mL; initial pH was used; initial concentration of CR = 10 mg L−1; catalyst dose = 1.0 g L−1.)
After Fig. 7 it is noticeable that the degradation efficiency of CR over TiO2–SA was much higher compared to that over TiO2. This result may be explained according the UV–Vis DRS spectra of pure TiO2 and TiO2–SA nanocomposite. As has been shown in Fig. 5, TiO2–SA absorbs more visible light than pure TiO2 due to its lower band gap energy. It is also remarkable that the degradation rate of CR became higher when the photocatalysts were supported on PT. The low activity of the non-supported photocatalysts is due to its deposition at the bottom of the reactor. On the other hand, the TiO2–SA/PT catalysts showed high activities. However, as the TiO2–SA content increases in the TiO2–SA/PT composite the photocatalytic activity decreases. The same tendency was observed with the TiO2/PT composites. This behavior may be due to the opacity caused by excess of the photocatalyst clusters. The presence of these clusters reduces light penetration and increases the scattering effect. Moreover, it leads to a very weak dispersion of the catalyst particles, a weak transfer material and a bad illumination.
Additionally, the maximum photocatalytic efficiency was achieved using TiO2–SA (50 wt %)/PT to remove about 98.2% of the CR from the solution. The efficient photocatalytic activity of the TiO2–SA (50 wt %)/PT floating photocatalyst under solar light irradiation resulted from the efficient illumination and oxygenation which are fundamental for the photocatalytic process. The optimization of illumination and oxygenation should result in higher rates of radical formation and oxidation efficiency.
The surface modification of TiO2 by SA is advantageous in the photocatalytic process for the following reasons: First, the small size of particles and subsequently the high specific surface area of TiO2–SA offer more adsorption sites and remarkably allow an increase of the local concentration of organic dye in the vicinity of the TiO2 photoactive layer [33]. Second, the TiO2–SA nanocomposite has a structure which could enhance the light-capturing efficiency by multiple reflections of light between the organic chelating ligands (SA). Third, the modification of the TiO2 surface by SA decreases the rapid recombination rate of the photogenerated electron−hole and the radiative recombination rate of the self-trapped excitons.
3.8 Effect of the photocatalyst loading
Fig. 8 shows the effect of the amount of TiO2–SA (50 wt %)/PT floating photocatalyst on the photodegradation efficiency of CR. It can be observed that as the amount of the photocatalyst increased the CR discoloration also increased. These results suggest that the TiO2–SA content in PT has an important effect on the reaction kinetics. In particular, if the amount of TiO2–SA (50 wt%)/PT was equal to 1.0 g·L−1, the CR discoloration attained after 150 min of solar light irradiation was 94.6% without any stirring or oxygenation.
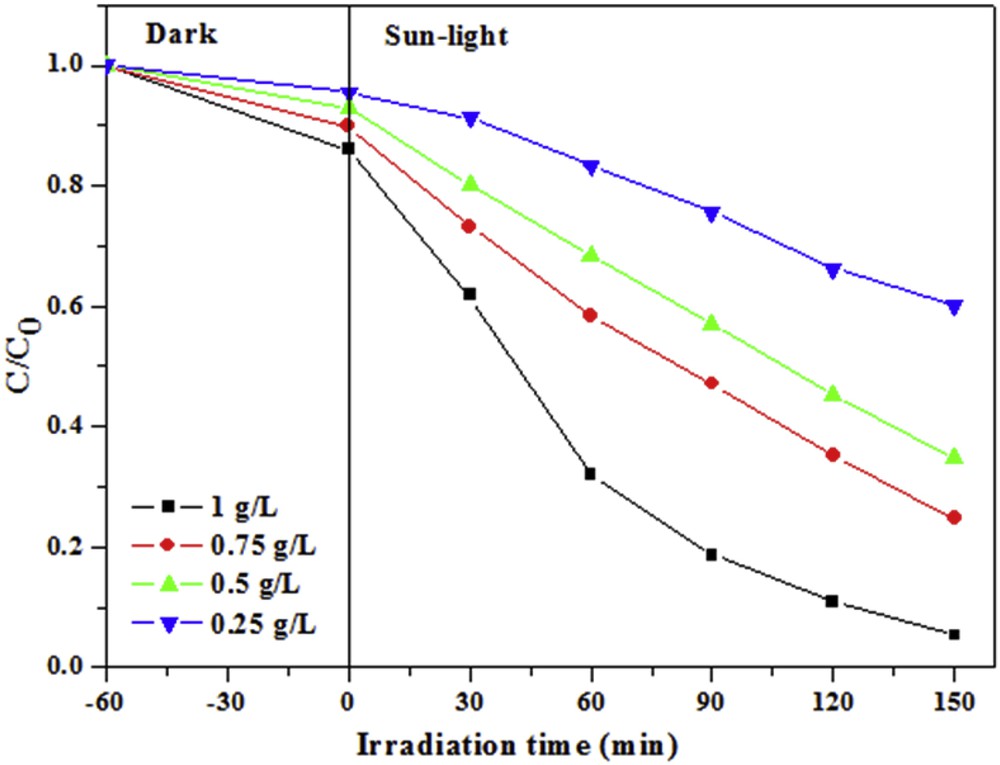
Effect of the amount of TiO2–SA (50 wt %)/PT floating photocatalyst on the discoloration of CR dye after 150 min. (Experiments were carried out under solar light irradiation, T = 34 ± 2 °C, volume = 100 mL; pH: initial; initial concentration of CR = 10 mg L−1.)
3.9 Effect of hydrogen peroxide
Hydrogen peroxide (H2O2) is an electron scavenger which may accept a photogenerated electron from the conduction band and thus prevents electron–hole recombination by forming •OH radicals [34]. For this reason, the effect of H2O2 dosage, from 5 to 35 mM, on the photodegradation of CR was investigated in the presence of TiO2–SA (50 wt %)/PT. As shown in Fig. 9, there was an optimal dosage of H2O2, 20 mM, at which the degradation efficiency of CR attained a maximum. Below the optimal dosage, the enhancement of the degradation efficiency by the addition of H2O2 could be attributed to the increase of reactive hydroxyl radical concentration [35]. The increase in the concentration of hydroxyl radicals resulting from the decomposition of H2O2 promoted the CR degradation process. Ohno et al. have reported that the interaction between H2O2 and TiO2 leads to the formation of titanium peroxide complex TiO2–H2O2 on the TiO2 surface. The TiO2–H2O2 complex extends the photoresponse of TiO2 to the visible region, leading to the visible-light-induced surface electron transfer from the surface complex to the conduction band of TiO2 [36]. The electrons on the conduction band of TiO2 initiate the decomposition of H2O2, which gives rise to the generation of hydroxyl radicals as follows [37]:
H2O2 + e− → •OH + OH− |
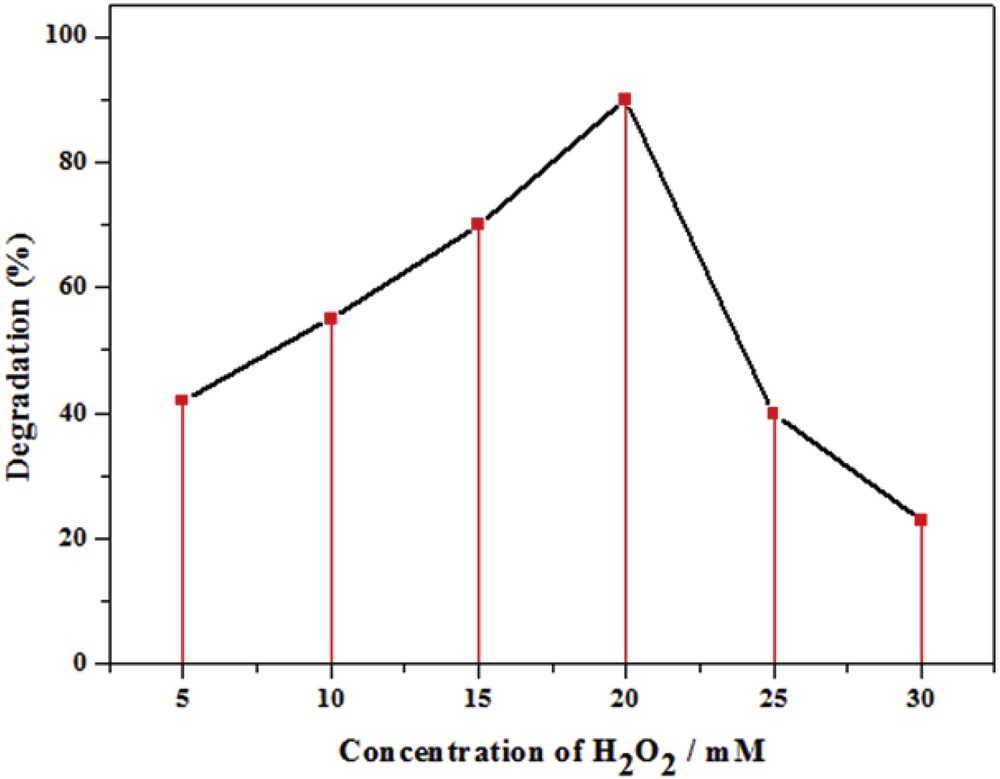
Effect of dosage of H2O2 on the photodegradation of CR after 100 min in the presence of TiO2–SA (50 wt %)/PT. (Experiments were carried out under solar light irradiation, T = 34 ± 2 °C, volume = 100 mL; pH: natural; initial concentration of CR = 10 mg L−1; catalyst dose = 1.0 g L−1.)
H2O2 is expected to play a double role in the photocatalytic degradation process. On receiving a photogenerated electron from the conduction band of TiO2, it promotes the charge separation and forms •OH radicals as follows:
H2O2 + O2 → OH− + •OH + O2 |
When H2O2 was added at higher dosage, the degradation efficiency of CR decreased. This was because the very reactive HO• radicals and the valence band holes could be consumed by H2O2 itself [38,39]. This negative effect of high concentration of H2O2 may be due to the formation of HO2•, which is significantly less reactive than HO•. Thus, H2O2 acts as a powerful HO• scavenger along with the photogenerated holes and inhibits the generation of HO• radicals and thereby decreases the degradation rate as follows:
H2O2 + •OH→H2O + HO2• |
HO2• + HO•→H2O + O2 |
H2O2 + h+→HO2• + H+ |
Therefore, the mechanism behind the photodegradation of organic compounds in the presence of the modified TiO2/H2O2 system is still not well defined. The effect of H2O2 addition on the kinetics of degradation is not always positive and seems to depend on the studied system.
3.10 Photocatalyst reuse
The ability of a photocatalyst to be reused is one of the most important parameters which determines, from an economical point of view, the potential exploitation of a material in practical systems for water treatment. To examine the repeatability of the photocatalytic activity, the TiO2–SA (50 wt %)/PT was used for four consecutive photodegradation cycles. The concentration of the organic compound (CR) was measured after 210 min of solar light irradiation (Fig. 10). After each cycle, the photocatalyst was rinsed, dried and used again under the same conditions.
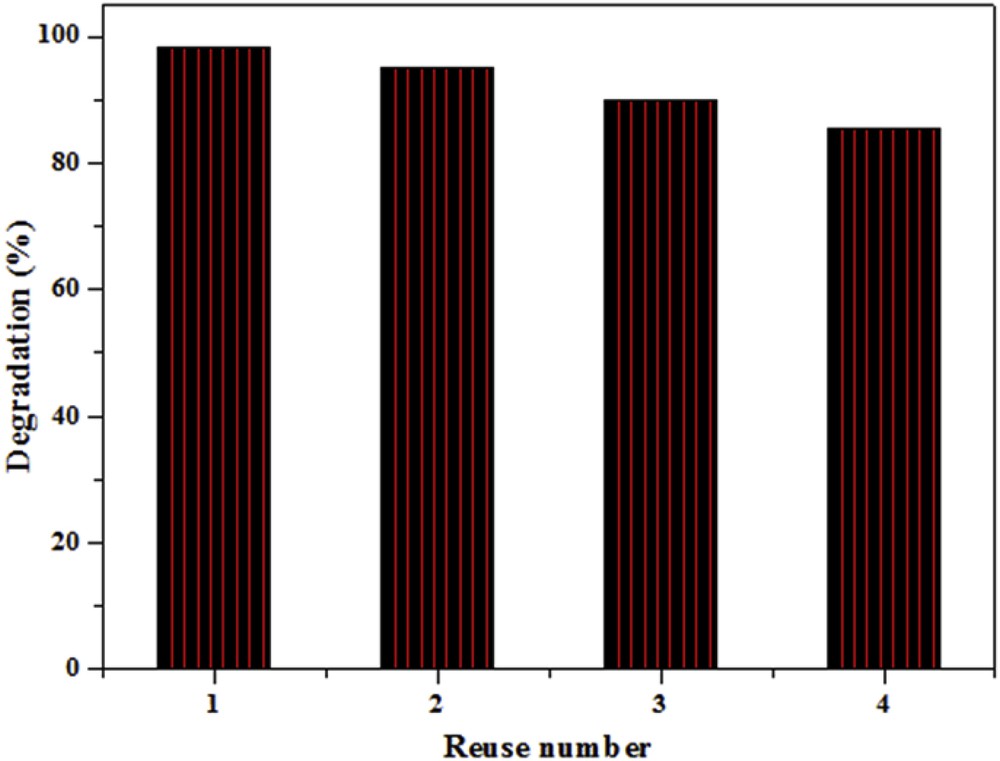
Effect of reuse number of TiO2–SA (50 wt %)/PT on the photodegradation of CR after 210 min of solar light irradiation. (Experiments were carried out under solar light irradiation, T = 34 ± 2 °C, volume = 100 mL; pH: natural; initial concentration of CR = 10 mg L−1; catalyst dose = 1.0 g L−1.)
As shown in Fig. 10, the photodegradation efficiency of CR exceeds 80% in the presence of TiO2–SA (50 wt %)/PT after four runs of solar light irradiation. On the other hand, the photocatalytic efficiency decreases after each cycle of treatment. The decreased performance in the degradation of CR may be explained by the formation of by-products and their accumulation on the active surface sites of the photocatalyst.
3.11 Photocatalytic tests with other pollutants
To evaluate the photoactivity of the TiO2–SA (50 wt %)/PT floating photocatalyst with organic pollutants other than CR dye, photodegradation experiments were performed with phenol, 4-nitrophenol, 2.4-dinitrophenol and toluidine. Fig. 11 shows that degradation rates of 58.4, 83.0, 70.7 and 61.6% could be attained for phenol, 4-nitrophenol, 2.4-dinitrophenol and toluidine, respectively. This result shows that the TiO2–SA (50 wt %)/PT floating photocatalyst has a wide applicability for the removal of organic pollutants from wastewater. Therefore, this novel type of floating photocatalyst will likely trigger a strong interest in this field of research.
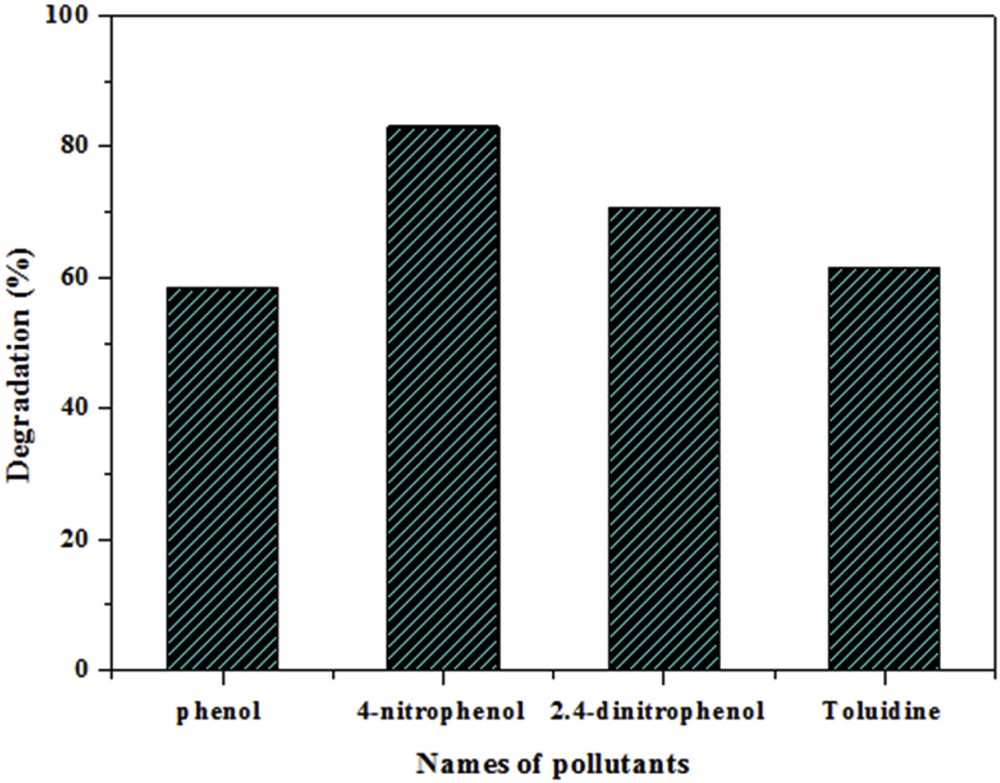
Photodegradation efficiency of different organic pollutants in the presence of TiO2–SA (50 wt %)/PT after 210 min. (Experiments were carried out under solar light irradiation, T = 34 ± 2 °C, volume = 100 mL; natural pH; initial concentration of pollutants = 10 mg L−1; catalyst dose = 1.0 g L−1.)
3.12 Possible visible-light-induced photocatalytic mechanism
According to the above experimental research, a probable mechanism for charge transfer and photocatalytic organic dyes over the TiO2–SA catalyst is suggested and illustrated in Scheme 2. The band gaps of TiO2 and SA deduced from Fig. 5 are 3.2 eV and 3.97 eV, respectively. The extension of the light absorption of TiO2–SA to the visible region may be attributed to the synergistic effect between the inorganic and organic composites [40]. The relative energy levels of SA (π and π* orbitals) and TiO2 (conduction (CB) and valence (VB) bands) are shown in Scheme 2. According to the experimental results, the mechanism of photocatalytic degradation under solar light irradiation may be interpreted as follows: As a semi-conductor material, SA absorbs visible light to induce π–π* electron transition. Afterward, the excited-state electrons are transported from the π-orbital to the π*-orbital. The energy levels of the TiO2 d-orbital (CB) and the SA π*-orbital match well for the charge transfer [12,41]. So, the excited-state electrons can be readily injected into the CB of TiO2 and transferred to the nanocomposite surface to react with oxygen and form superoxide radicals •, subsequently. At the same time, a positive charged hole (h+) might be formed by electron migration from the TiO2 valence band to the SA π-orbital, which can react with H2O in the solution to generate •OH. The superoxide radical ion • and hydroxyl radical •OH are responsible for the degradation of organic compounds. The whole process is shown and described in Scheme 2.
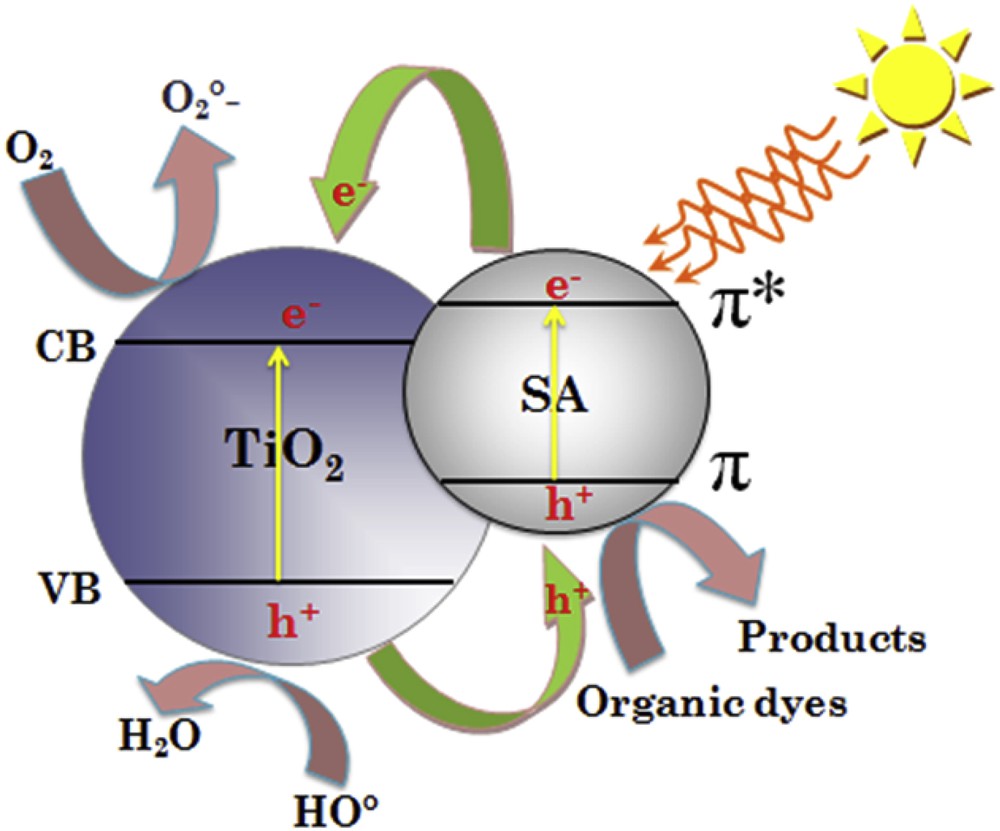
Mechanism of electron−hole migration under sunlight (e−: electron; h+: hole).
Therefore, the whole visible-light-induced photocatalytic process of TiO2–SA can be summarized briefly as follows:
(1) |
(2) |
(3) |
(4) |
(5) |
(6) |
(7) |
Subsequently, large numbers of •OH and superoxide (•) radicals were generated by the decomposition of water (Eq. (3)) and in the presence of O2 (Eq. (4)). • may form organic peroxides in the presence of organic scavengers (Eq. (5)). While holes in the photocatalyst allow the direct oxidation of CR to reactive intermediates (Eq. (6)) [42], the •OH radicals allow the degradation of CR to form final decomposition products (Eq. (7)). The recombination process of the electron−hole pairs is then suppressed, and the charge separation and stabilization are reached. Consequently, the efficient electron−hole separation leads to a remarkable enhancement of photocatalytic CR degradation in the presence of TiO2–SA composite.
4 Conclusion
The results presented in this work have shown that PT can be used to support TiO2–SA to prepare an active floating photocatalyst. The PT is a natural material, non-toxic and low cost. As a catalyst support, PT offers a good chemical, thermal and mechanical resistance. On the other hand, the obtained results showed that surface modification of TiO2 nanoparticles by SA via the impregnation method is a facile way to improve its photocatalytic activity under solar light irradiations. The prepared TiO2–SA/PT floating photocatalyst can be used to remediate waters contaminated with dyes, phenols or other pollutants. It can be recovered and recycled at least four times before losing high activity. The floating photocatalysts have potential applications, such as the treatment of contaminated wastewater reservoirs located in remote areas without any special equipment or installation. In particular, they can be used for the destruction of suspended insoluble organic contaminants, e.g., in oil-spill accidents.