1 Introduction
In recent years, metallic nanoparticles are among the intensively studied nanomaterials, because of their high surface area and chemical, electronic, thermal, optical, magnetic, catalytic, and biological properties [1–3] that are different from those of their bulk form. Because of the nanostructural effect, numerous nanomaterials are applied in catalysis, medical instrument, and device, water treatment, food processing, and antimicrobial applications. Among the metal-based nanoparticles, copper nanoparticles (CuNPs) have received special attention because of its useful properties achievable at much lower cost and abundance than the well-known and used silver or gold nanoparticles. Copper compounds have been used widely in agricultural practices as trace element nutrition, fungicides, pesticides, algaecides, and herbicides as well as in animal husbandry as a disinfectant [4]. Many reviews on the preparation of CuNPs and their various applications have been also published, reporting ideal characteristics such as high electrical conductivity [5] lubricative, thermal [6], and antimicrobial antifungal/antibacterial activities [7]. As a matter of fact, the antimicrobial activity of CuNPs has been reported to be stronger than that of copper salts because of their unique property, i.e. large surface-to-volume ratio and crystallographic surface structure [8]. CuNPs have strong inhibitory and antifungal activities for a broad spectrum of fungi [3,4,9,10].
In recent years, synthesis and utilization of CuNPs as antifungal agent have been receiving more attention because of gradual increase of drug resistance among microorganisms [4]. Cao et al. [3] investigated the antifungal activity of CuNPs against Corticium salmonicolor, which causes pink disease on rubber and wood trees in tropical regions. The results revealed that a colloidal of 7 ppm CuNPs exhibited powerful antifungal activity. Jeyaraman et al. [11] reported the promising antifungal activity of CuNPs against three fungal strains of Aspergillus flavus, Aspergillus niger, and Candida albicans. Moreover, the CuNPs also exhibited the promising antifungal agent against plant pathogenic fungi Fusarium oxysporum, Curvularia lunata, Alternaria alternata, and Phoma destructiva [4].
Many researches have been reported to synthesize CuNPs by using special precursors such as copper acetate [7], copper(II) nitrate [4,12], hydrazine dihydrate [3]. Synthesis of CuNPs using special precursors may involve chemicals harmful to human health and environment [13] or lead to increasing production cost. Hence, it is necessary to synthesize CuNPs using effective and economical technologies for agricultural applications.
Ascorbic acid (also commonly known as “vitamin C") has been used both to reduce metals cation precursors and to prevent oxidation the metallic nanoparticles. Moreover, in term of agricultural applications, ascorbic acid is considered as an antioxidant, enzyme cofactor, and electron transport in plants [14]. Ascorbic acid plays a significant role to protect against an activated form of O2 for N2 fixation in root nodules of legume plants [15]. It is known that antioxidant activities of ascorbic acid might contribute to alleviating the adverse effects of water deficit on plant growth, yield, and irrigation water use efficiency [14]. Moreover, Aziz et al. [16] recorded remarkable enhancement in reducing the damaging action of drought and decreased enzyme activity because of the scavenging of reactive oxygen species by applying ascorbic acid. Consequently, it may reduce the adverse effects of drought stress in quinoa plants or maize plants in arid regions [16]. Taking advantages of ascorbic acid in chemical reduction agent and agricultural applications, ascorbic acid is essentially used to synthesis CuNPs for evaluating of its antifungal activity.
Plant pathogenic fungi, that are responsible for the destruction of a large number of plants, cause huge economic losses to agricultural production [17,18]. F. oxyporum and Phytophthora capsici (P. capsici) are known as the causal agent of wilt that causes considerable crop yield loss [19–21]. Up to now, there have been almost no studies on the antifungal activity of CuNPs, which are produced via green chemical route, against F. oxyporum and P. capsici.
The stabilizers of colloid are also an important factor to control their stability for application [22]. In recent years, the synthesis of CuNPs in a reasonable polymer matrix has been much attention. Among various candidates polymers used for synthesizing nanoparticle such as amines, thiols, and alcohols [23], polyvinylpyrrolidone (PVP) is revealed as a promising stabilizing agent. PVP protects the nanoparticles from aggregation by steric stabilization and by ligandization of nanoclusters surface atoms [23,24]. However, amount of PVP, one of the critical factors, which may affect the formation of Cu particles, is needed to be studied.
Moreover, synthetic conditions such as solution pH, molar ratio of the reactants, reaction time, and ratio of PVP play an important role in the size and structure of nanoparticles, which strongly affects the properties of CuNPs, on which most of the industrial applications of CuNPs are based. Consequently, copper as a nanomaterial is engineered to obtain nanosizes so as to enhance their antifungal activities. In this study, a series of experiments were carried out to investigate the factors influencing the size of the copper samples. The effects of particle size and concentration on the antifungal activities of CuNPs against the selected crop pathogenic fungi F. oxysporum and P. capsici were also evaluated.
2 Experimental
2.1 Materials
Analytical grade precursor salt CuCl2·2H2O (99.98%), NaCl (99.5%), HCl (37–38 %), ascorbic acid (99.0%), and PVP (Mw = 40,000 g/mol) were supplied by Merck and used as received without further purification. Deionized water was used throughout the experiments.
2.2 Synthesis of CuNPs
CuNPs were synthesized by the reduction of copper(II) chloride with ascorbic acid in the presence of the surfactant PVP via the following method as shown in Fig. 1. A typical 40 mL 0.04 mol/L of copper chloride solution was prepared in a 250 -mL three-neck round-bottom flask equipped with a water-bath and a thermometer. Varying amounts of PVP was dissolved into the prepared copper chloride solution. The mixture was stirred and kept at room temperature. Whereas, a 40 mL ascorbic acid solution with different concentrations was also prepared. Both solutions were bubbled with nitrogen gas for 1 h, and the solution pH values were adjusted to the same value of 3, 5, 7, 9, or 11 by adding appropriate amount of aqueous NaOH solution (0.1 mol/L) or H2SO4 solution (0.1 mol/L). Ascorbic acid solution was then added drop-wise to the copper mixture with vigorous stirring. The mixture was then heated and kept at around 80°C for 1, 2, 3, or 4 h to carry out the reduction reaction. In this synthetic process, the color of the mixture gradually changed from milky white to yellow, orange, and finally brick red. Nitrogen gas bubbling was stopped when the reaction system was cooled down to room temperature. The colloidal CuNPs were transferred into pyrex vail and then bubbled with nitrogen for 1 h to estimate the saturated nitrogen concentration to prevent oxidation during storage for further analysis.

Flowchart of preparation of Cu nanoparticles.
The solution for negative control test (controlled solution) was prepared in the same manner of preparation of CuNPs that reduction temperature at 80°C for 3 h, pH 7, ascorbic acid:Cu2+ molar ratio 5:0 (using 40 mL of distilled water instead of 40 mL 0.04 mol/L of copper chloride solution) and 12.8 g PVP (with accordant to the optimal condition that the weight percentage of Cu2+ over PVP is 1%), but without any copper(II) chloride agent. Moreover, to compare the antifungal activity of CuNPs to Cu2+ cations, a 40 ppm CuCl2 solution was made by dissolving CuCl2 in deionized water.
2.3 Characterization
Ultraviolet-visible (UV–Vis) absorption spectroscopy is a well-known tool to determine the formation of CuNPs that exhibit the surface plasmon resonance (SPR) peak in a 550–650 nm range [25]. Therefore, in this research, the UV–Vis spectrum of CuNPs was recorded from 450 to 750 nm by a U-2910 spectrophotometer of Hitachi, Japan. The crystallinity and phase composition of the generated CuNPs were investigated by using a D8 Advance-Brucker X-ray diffractometer using CuKα radiation with the wavelength λ =1.5406 Å. The X-ray diffraction (XRD) pattern was recorded in the 2θ range from 20° to 80° at scanning steps of 0.02°. The morphology of obtained nanoparticles was observed by a transmission electron microscope (TEM, JEOL-JEM1010, Japan).
2.4 Antifungal activity
The tested fungal F. oxysporum and P. capsici were obtained from Plant Disease Control Center II, Vietnam. Antifungal activity of the CuNPs against F. oxysporum and P. capsici was studied by the agar disc diffusion method using potato dextrose agar (PDA). F. oxysporum and P. capsici were cultured on PDA at 25°C in the dark. CuNPs were dispersed in a PDA media at different concentrations and then autoclaved before pouring into the Petri dishes (9 cm diameter). The fungi were inoculated after the PDA media solidified at 25°C. A 4-e mm diameter well was made on the surface of each agar PDA plate using a sterile cork borer. The well on each plate was filled up by the same amount of each fungus. The inoculated plates were incubated at 30°C for 2 or 5 days for P. capsici or F. oxysporum, respectively. The zone of inhibition (mm) of P. capsici was measured by a Vernier caliper after 1 and 2 days of incubation, while it was recorded of F. oxysporum after 3 and 5 days of incubation. The growth inhibition (GI) zone of fungus was calculated using the following formula:
(1) |
3 Results and discussion
3.1 Physical properties
3.1.1 Effect of the solution pH
The effect of the solution pH on the size of CuNPs at a reaction temperature of 80°C and a reaction time of 3 h was investigated. The UV–Vis spectra of colloidal CuNPs solutions, prepared under various pH levels, are presented in Fig. 2(a). As can be seen from Fig. 2(a), the pH of solution strongly affects the SPR of CuNPs. Copper particles which were synthesized at pH 3, 5, and 7 display a broad absorption peak at approximately 600 nm (pH 3), 590 nm (pH 5), 584 nm (pH 7), respectively. These results revealed that the pH of solution affects the formation and particles size of CuNPs. When the pH of solution was increased from 3 to 7, the absorption peak was found to shift from 600 nm to 584 nm. The blue shift toward the shorter wavelength was attributed to decreasing particle size. According to Wu [26], the potential decreases with an increasing pH value of solution, leading to increase in reduction power of ascorbic acid. A higher reduction power of ascorbic acid make to increase reaction rate resulting a large amount of copper nuclei. Consequently, a small particle of CuNPs was obtained [26,27]. Moreover, absorption peak was not observed at pH 9 and pH 11, revealing that basic medium prevented the formation of CuNPs. This effect might be because of the fact that ascorbic acid is unstable and oxidized rapidly at pH > 7 [28]. Therefore, the Cu2+ cation could not be reduced to Cu° at pH > 7.
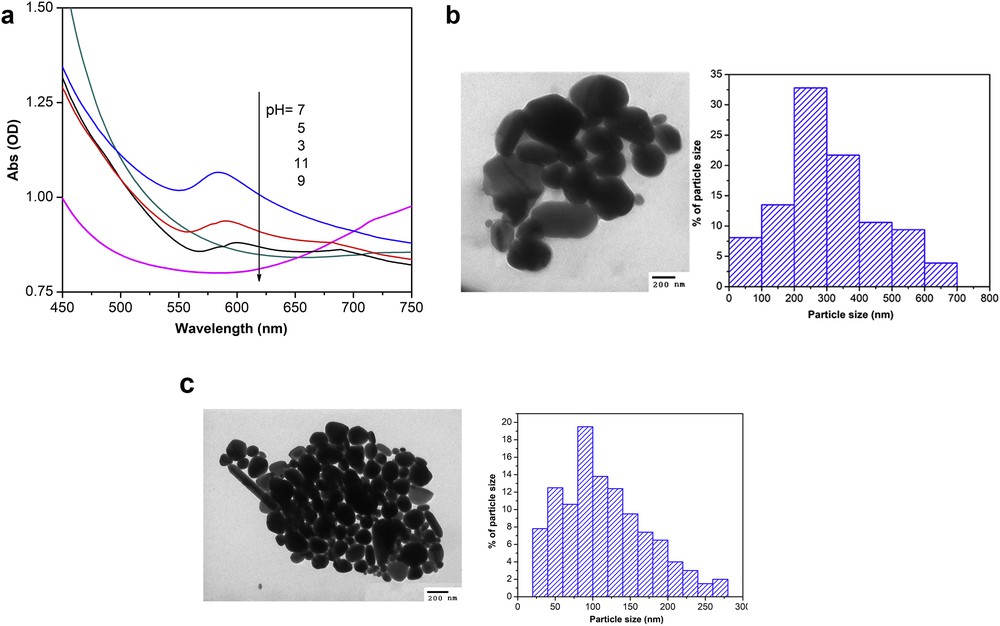
(a) UV–Vis spectra of colloidal CuNPs solutions, prepared under various pH levels, and TEM images and the particle size distribution of synthesized CuNPs at (b) pH 3 and (c) pH 7. Other operation conditions synthesized at 80°C for 3 h, ascorbic-acid-to-Cu2+ molar ratio 5:1, weight percentage of Cu2+ over PVP 7%.
The effect of pH on the particle size of CuNPs was also confirmed in the TEM images. The TEM micrographs and size distribution of colloidal CuNPs which were produced at pH 3 and pH 7 for 3 h are presented in Figs. 2(b) and (c), respectively. As can be seen from Figs. 2 (b) and (c), the produced CuNPs mainly consisted of nearly spherical particles whose measured diameter is 336 nm on average at pH 3 and 120 nm on average at pH 7. The recorded images clearly demonstrate that when pH was increased, the size of copper particles produced was found to decrease dramatically. Consequently, small particles size of CuNPs could be synthesized at increasing the pH value of reaction mixtures which is in good agreement with the literatures [25,29–31].
3.1.2 Effect of reduction time
As reported in the literature, ascorbic acid is a mild reducing agent; the rate of reduction of Cu2+ is slow. The reduction reaction of Cu2+ to form Cu particles at pH 7 takes place in the two following steps [32]:
Cu2++ 2OH−→ Cu(OH)2 | (2) |
Cu(OH)2+ C6H8O6 → Cu + C6H6O6 + 2H2O | (3) |
It is therefore necessary to investigate the effect of reaction time (after the addition of ascorbic acid) on the formation of CuNPs from the reduction of Cu2+ at pH 7. A series of experiments were conducted at various reduction times with pH 7, ascorbic-acid-to-Cu2+ molar ratio 5:1, reaction temperature of 80°C. Fig. 3 shows UV–Vis spectra of obtained colloidal samples when the chemical reduction proceeded at pH 7 for 1 h, 2 h, 3 h, and 4 h. As seen from Fig. 3, copper particles display absorption peaks at reduction time of 2 h, 3 h, and 4 h, but not at 1 h reaction, revealing that copper particles are formed after proximately 2 h. On the other hand, the sharp and narrow absorption peaks are found at 3 h (584 nm) and 4 h (586 nm) reaction, which can be attributed to absorption by nanosize copper particles. Moreover, the intensity of absorption peaks increased with increased reaction time (from 2 to 3 h) and got stable after 3 h revealing the stability of produced CuNPs. This trend suggests that there are no more Cu particles formed at reaction times greater than 3 h.

UV–Vis spectra of colloidal CuNPs solutions synthesized at 80°C, ascorbic-acid-to-Cu2+ molar ratio 5:1, weight percentage of Cu2+ over PVP 7%, pH 7, and under various reduction time.
3.1.3 Effect of the ascorbic-acid-to-Cu2+ molar ratio
It is known that molar ratio of the reactants (ascorbic-acid-to-Cu2+ molar ratio) strongly affects efficiency and particle size of CuNPs. Fig. 4(a) shows the UV–Vis spectra of CuNPs samples produced at pH 7, 80°C for 3 h with various molar ratios of reactants (5:1, 10:1, 20:1, and 40:1). Absorption peaks of CuNPs which were synthesized at molar ratio of reactants of 5:1, 10:1, 20:1, and 40:1 display at approximately 576, 581, 584, and 591 nm, respectively. The red shift in SPR peak position when increasing amount of ascorbic could be attributed to the increase in particle size. A high molar ratio of reactants lead to dramatically increase of the rate of chemical reduction, rapid formation of a large number of copper nuclei, and forming very small particles, resulting in the increased aggregation of copper atoms and particles. In addition, when the molar ratio of reactants was larger than 10 (i.e. 20:1 and 40:1), absorption peaks were found to indicate a decrease of absorption intensity and a broadening. Evidently, Figs. 4(b) and (c) show a different particle size distribution of produced CuNPs. Therefore, the samples that were synthesized at pH 7, a reduction temperature of 80°C for 3 h, and a ascorbic acid:Cu2+ molar ratio 5:1 showed the smallest particles size.
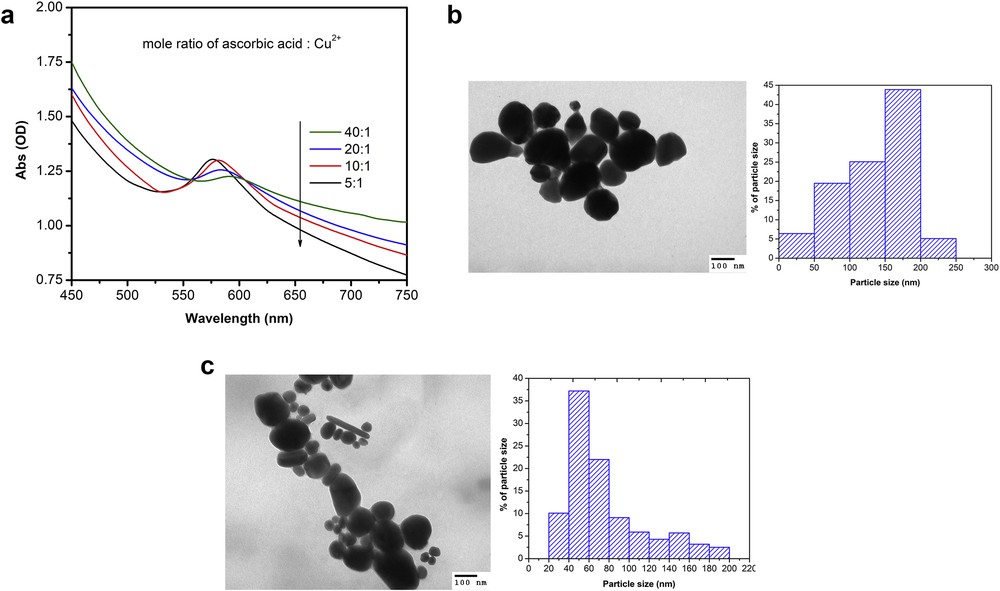
(a) UV–Vis spectra of colloidal CuNPs solutions synthesized at pH 7, 80°C for 3 h with various molar ratios of reactants, and TEM images and particle size distribution of synthesized CuNPs at a ascorbic-acid-to-Cu2+ molar ratio (b) 40:1 and (c) 5:1.
3.2 The protection of PVP on the copper nanoparticles
In this research, the effect of the amount of PVP on the formation of CuNPs was studied. UV–Vis absorption spectra (Fig. 5(a)) of the CuNPs synthesized at pH 7, 80°C for 3 h, molar ratio of reactants 5:1 with various weight percentage of Cu2+ over PVP of 1%, 3%, 5%, and 7% are found in Fig. 5(a). It can be seen that a broad absorption peak of samples at different weight percentage of Cu2+ over PVP of 1%, 3%, 5%, and 7% was observed at 567, 570, 576, and 584 nm, respectively. The absorption peak shifted from 567 nm to 584 nm when weight percentage of Cu2+ over PVP was increased. The red shift in SPR peak position suggests that the size of the generated CuNPs was increased. The average of CuNPs size of samples was measured at 53 nm (1%), 84 nm (3%), 120 nm (5%), and 174 nm (7%). The recorded images (Figs. 5(b) and (c)) clearly confirmed the relationship between decreasing particles size of CuNPs with increasing amount of PVP. In other words, the present of PVP in larger amount may fully protect the CuNPs from growing and agglomerating [33] and may avoid copper oxide formation [7] because of form coatings on the surface of CuNPs. It can be summarized that the smallest size of CuNPs could be synthesized under conditions such as pH 7, reduction temperature of 80°C for 3 h, ascorbic acid:Cu2+ molar ratio 5:1, and weight percentage of Cu2+ over PVP 1%.
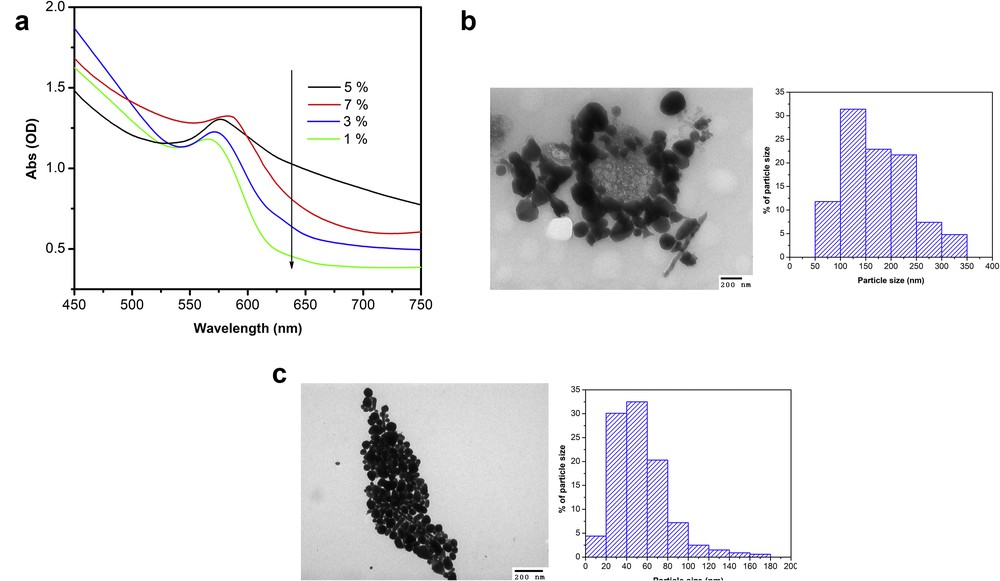
(a) UV–Vis adsorption spectra of colloidal CuNPs solutions synthesized at various PVP level (weight percentage of Cu2+ over PVP), and TEM images and particle size distribution of synthesized CuNPs at weight percentage of Cu2+ over PVP (b) 7% and (c) 1%. Other operation conditions synthesized at 80°C for 3 h, ascorbic-acid-to-Cu2+ molar ratio 5:1 and pH 7.
3.3 Structural analysis
The crystal structure and phase composition of the CuNPs with the smallest size were analyzed by XRD method. Fig. 6 shows the XRD patterns of the sample. The X-ray diffraction peaks, which appeared at a 2θ value of 43.2° (111), 50.4° (200), and 74.1° (220) are well indexed to the JCPDS card No. 04–0836 representing the face-centered cubic structure of copper. The sharp and strong diffraction peaks revealed that CuNPs are highly oriented. Moreover, no characteristic peaks of oxide impurities could be detected confirming that the obtained CuNPs are single phase.
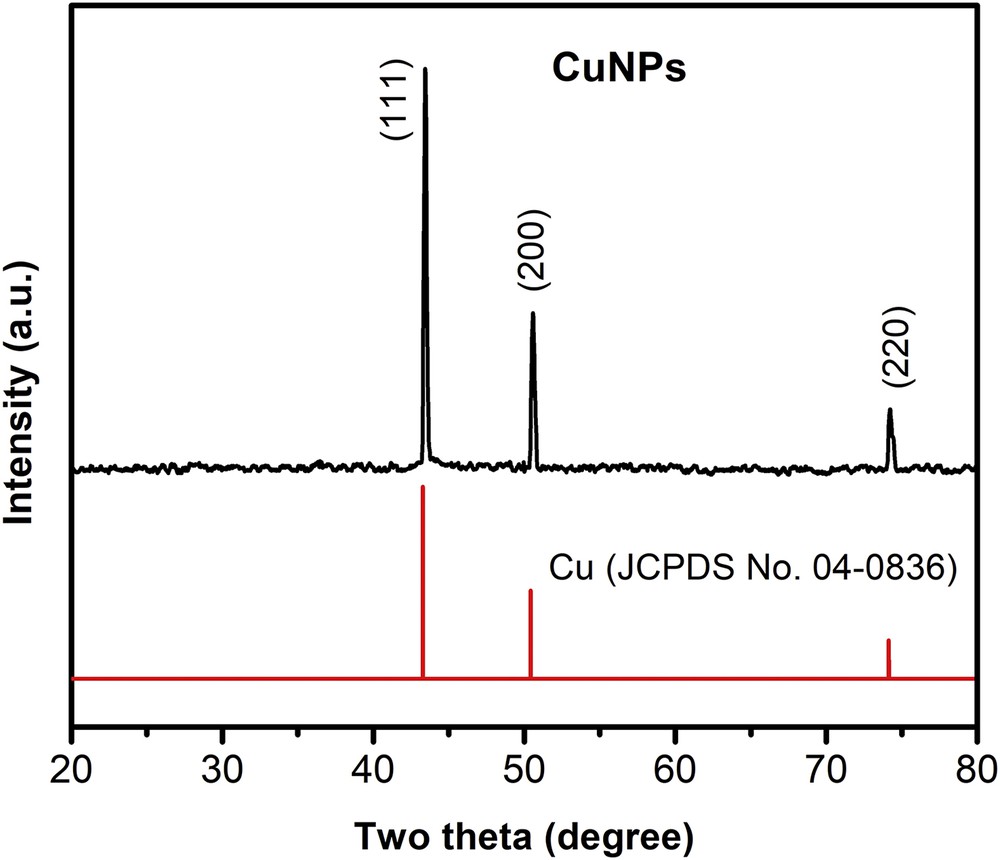
The XRD pattern of the synthesized Cu nanoparticles synthesized at pH 7, 80°C for 3 h, ascorbic-acid-to-Cu2+ molar ratio 5:1, and weight percentage of Cu2+ over PVP 1%.
3.4 Antifungal activity
The colloidal solutions of CuNPs at various concentrations (5, 10, and 20 ppm) with the different particle sizes including 174 nm, 120 nm, 84 nm, and 53 nm were used to evaluate the inhibitory activity against fungus versus time of treatment. Figs. 7(a)–(e) provide the effect of particle size, concentration, and incubation time on the antifungal activity of CuNPs against the fungus of F. oxysporum and P. capsici. In general, the growth inhibition of CuNPs against both F. oxysporum and P. capsici increased with increasing incubation time of treatment and/or with decreasing particle size. In terms of F. oxysporum inhibition, when increasing concentration of CuNPs from 5 ppm to 10 ppm and 20 ppm, even at different particle size, growth inhibition slightly increased. For instance, by using particle size of 174 nm, growth inhibition, measured after 3 days (Fig. 7(a)), increased from 17% to 33%–49% when increasing concentration from 5 to 10–20 ppm, respectively. Moreover, the growth inhibition increased with increasing time of treatment. The highest growth inhibition was obtained at 20 ppm by using the smallest particle size of 53 nm, achieving at approximately 63% after 3 days (Fig. 7(a)), 66% after 5 days (Fig. 7(b)), and 72% after 7 days (Fig. 7(c)).

GI of CuNPs at various particle sizes (174, 120, 84, and 53 nm) against F. oxysporum after (a) 3 days, (b) 5 days, and (c) 7 days of incubation and against P. capsici after (d) 1 day and (e) 3 days of incubation.
Besides, all CuNPs samples that were also prepared at 5 ppm, 10 ppm, and 20 ppm concentrations with different particles sizes showed highly inhibited activity against P. capsici (Figs. 7(d)–(e)). The growth inhibition over P. capsici dramatically increased with increasing concentration of CuNPs and decreasing particle size. Especially, four samples with different particle sizes which were prepared at 20 ppm showed complete inhibition the growth of this fungus on the first day of incubation. Moreover, CuNPs at a lower concentration (10 ppm) with particle size of 84 nm also performed the extremely high inhibitory activity against P. capsici which showed complete inhibition on the growth of this fungus on the second day (Fig. 7(e)).
In conclusion, the CuNPs sample with the smallest particle (53 nm) exhibited the best antifungal activities in comparison with other samples. The superior in the growth inhibition of CuNPs with the smallest particle (53 nm) against F. oxysporum and P. capsici is because of the smallest size that can easily penetrate the cell membranes through its surface [34]; hence, the smaller particle size CuNPs show the highest growth inhibition activity than other samples.
Preliminary studies (as shown above) have presented antifungal activities of synthesized CuNPs. To get precise data in antifungal activity of CuNPs, the effect of concentration on the antifungal activity of CuNPs against F. oxysporum and P. capsici was carefully studied. In this part, we prepared CuNPs colloid with particle size about 53 nm in various concentrations from 1.25 ppm to 40 ppm, CuCl2 solution (40 ppm), and control solution. Moreover, three reiterations of each test were carried out. The results show that the growth inhibition over F. oxysporum increased with increasing concentration of CuNPs and achieved 100% at 30 ppm after 3 days of incubation (Fig. 8(a)). As shown in Fig. 8(a), the control solution had a slight inhibitory effect on F. oxysporum (around 0.4% after 7 days). This means that control solution would not enhance the antifungal activity.

Antifungal activity of CuNPs with particle size about 53 nm at various concentrations against (a) F. oxysporum and (b) P. capsici.
Similarly, the growth inhibition of CuNPs over P. capsici was also studied with various concentrations of CuNPs, as shown in Fig. 8(b). The growth inhibition over P. capsici has the same trend with F. oxysporum, increased with increasing concentration of CuNPs from 1.25 ppm to 15 ppm. The results show that P. capsici was completely inhibited after 1 day at 7.5 ppm. This means that at lower concentration (7.5 ppm), the colloidal solutions of CuNPs would enhance the antifungal activity to P. capsici. As mentioned previously, the antifungal activity of CuNPs against the P. capsici was stronger than that of the F. oxysporum. Moreover, the results of negative control test using control solution (only 3% after 2 days) also suggest that control solution would not enhance the antifungal activity, and CuCl2 solution (40 ppm) partly inhibited the growth of P. capsici. In conclusion, the results of the fungal growth inhibition tests indicate differences in the sensitivity of the F. oxysporum and P. capsici against the sample tested, with P. capsici being the most inhibited [18,35]. Difference in the sensitivity of the F. oxysporum and P. capsici might be because of the presence of complex cell wall with rigidity or their resistance to toxic chemicals [36].
4 Conclusion
The pure CuNPs were successfully synthesised using CuCl2 as the precursor, ascorbic acid as reducing agent, and the PVP as capping polymer. The formation of the obtained CuNPs, as well as their structure and morphology, were characterized by XRD, UV–Vis, and TEM. The results demonstrated that solution pH, reaction time, ascorbic-acid-to-Cu2+ molar ratio and concentration of PVP played crucial roles either in the formation or in tuning the size and morphology of CuNPs. In addition, the antifungal activities of synthesized CuNPs against F. oxysporum and P. capsici were also investigated. CuNPs could inhibit entirely the growth of F. oxysporum at 30 ppm after 3 days and P. capsici at 7.5 ppm after 1 day of incubation. The antifungal activity of obtained CuNPs against P. capsici was stronger than that of the F. oxysporum.
Acknowledgements
This research is funded by Ho Chi Minh City University of Technology, VNU-HCM, Vietnam, under grant number BK-SDH-2020- 1770444.