1. Introduction
Urinary tract infections (UTI), ranging from uncomplicated cystitis to severe pyelonephritis and nephrolithiasis, are the third most common type of infection in human medicine (after respiratory and alimentary infections), affecting 150 million people each year worldwide [1, 2]. As reported by Flores-Mireles et al. [3], the societal costs of these infections, including health care costs and time missed from work, are approximately US$3.5 billion per year in the United States alone.
Regarding clinical presentation, UTI is associated with flank or abdominal pain (70%), typical renal colic (rare), fever (26%), gross haematuria (18%), and sepsis (1%), but can be asymptomatic (8%—incidental diagnosis). Infection of the urinary system may lead to the formation of concretions [4, 5, 6, 7, 8, 9] as well as an incrustation of JJ stents [10, 11, 12, 13]. It is well known that infection stones form secondary to urease-splitting organisms such as Proteus, Klebsiella, Pseudomonas, or Staphylococcus, among others. With elevated urine pH due to infection of the urinary tract, the patient becomes prone to form magnesium ammonium phosphate hexahydrate (MAP or struvite), carbonated calcium phosphate apatite (carbapatite or CA), or amorphous carbonated calcium phosphate (ACCP) stones [14, 15].
It is worth noting that while infection can initiate the stone, it can also contribute to the progression of a pre-existing metabolic stone: in practice, the reality is undoubtedly more complex. Letavernier [16] suggests that a urinary metabolic anomaly such as hypercalciuria could favour crystallization of calcium phosphate in the presence of non-ureasic bacteria like Escherichia coli. It is a new concept in addition to classical situations described by Miano et al. [17] which consider two different clinical pictures: stones that develop following UTI, and stones complicated by UTI (stones with infection) which are metabolic stones that passively trap bacteria from coexistent UTIs and may or may not contain calcium.
This short review provides an overview of infection kidney stones (IKS) and treats both clinical and chemical data. We will start by considering epidemiologic data which indicate a significant increase in the prevalence of IKS. Thanks to the data bank from the Laboratoire des Lithiases of Assistance Publique-Hôpitaux de Paris which details the chemical composition of kidney stones determined by Fourier Transform InfraRed (FTIR) spectroscopy [18, 19] as well as clinical data from more than 85,000 patients, we will establish a significant relationship between UTI and different chemical compounds namely MAP, CA, ACCP and whitlockite (Wk). Information on the pathogenesis of IKS as well as the nature of the bacteria identified in the patients concludes this section. To complete this clinical description, the crystallographic structure and chemistry of three chemical compounds, namely MAP, CA and Wk, are presented, concluding with descriptions of crystallite morphology of these three chemical phases in the presence or absence of bacteria.
2. Clinical data regarding IKS
2.1. Some epidemiological data
In developing countries, major differences in the incidence of infection stones have been observed depending on continent and region, from 2.7% in Asia Minor to 13% in South America and 42.9% in Sub-Saharan Africa. These differences reflect the infectious risk factors specific to certain populations, such as nutrition and availability of modern medicine and antibiotics in each specific area [20].
Considering all criteria from stone analysis suggestive of infection-related stones, we and others [18, 21, 22] in industrialized countries observed a decrease of infection stones over several decades before 2000, but a constant increase in the proportion of stones related to infection in both sexes thereafter (Figure 1). Such a significant increase may be due to different factors such as the evolution of bacteria resistant to antibiotics, or more limited access to medicine. Of note, the relative proportion of UTI stone occurrence in men ranged from 3.2 to 10.1% and was slightly higher at the extremes of life [21]. As shown in Figures 1A and B, infection stones occur more frequently in female than in male patients across all ages.
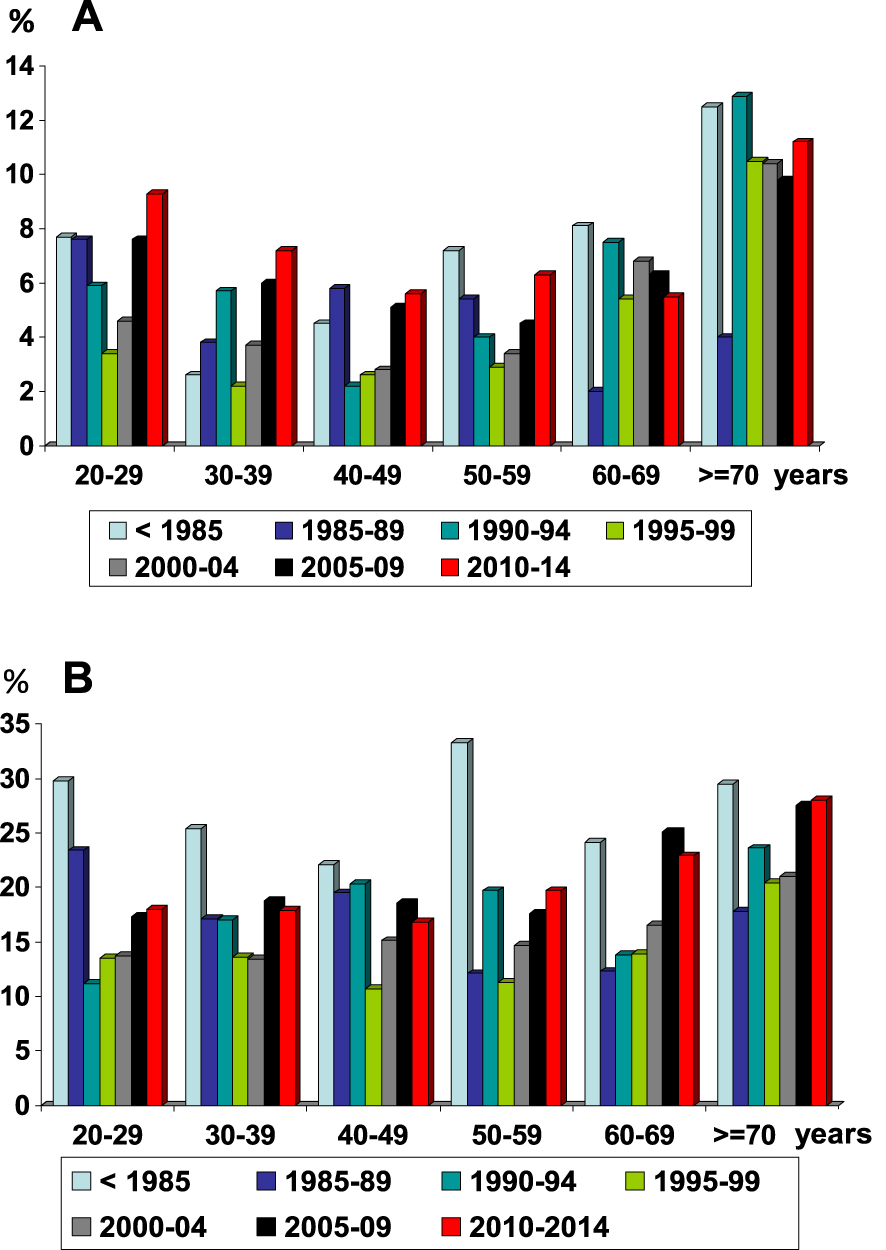
Evolution of infection stones in humans as a function of age and time period: A = men; B = women (from Ref. [18]). After a constant decrease in the proportion of infection stones in the second part of the twentieth century, we observed a new increase of infection stones at the beginning of the twenty-first century.
2.2. Chemical phases related to clinically indicated UTI
Each stone sent for analysis in our lab is accompanied by an information sheet including anthropometric and clinical data. A clinically symptomatic urinary tract infection is one of the queries. Based on the data bank, the relationship between UTI and the main chemical compound present in the chemical composition of the stones removed from patients is summarized in Table 1. Consistent with the literature, the first compound in Table 1 is struvite, considered as a strong marker of this lithogenic process [23, 24, 25, 26, 27, 28, 29, 30]. We note surprisingly that UTI was clinically identified in only 65.8% of cases where the stone contains any proportion of struvite. The link is slightly stronger for stones mainly composed of struvite (71.2%).
Relationship between UTI and the chemical composition of stones determined by FTIR spectroscopy
Main crystalline or amorphous phase | Number of UTI∗ | Number of patients without recognized UTI | Occurrence of UTI (%) |
---|---|---|---|
Struvite | 477 | 193 | 71.2d |
Wk | 35 | 31 | 53.0a,c,e |
ACCP | 21 | 20 | 51.2a |
CA with CO3 rate ⩾ 15% | 354 | 323 | 52.3a,d |
OCP | 21 | 42 | 33.3 |
CA (overall) | 1024 | 2276 | 31.0b |
AmUr | 27 | 62 | 30.3b |
Brushite | 160 | 579 | 21.7g |
UA0 | 542 | 2558 | 17.5 |
CYS | 89 | 427 | 17.3 |
UA2 | 47 | 402 | 10.5f |
COM | 1900 | 16,675 | 10.2 |
COD | 613 | 7148 | 7.9 |
Total | 4956 | 30,413 | 14.0 |
∗UTI was defined as UTI clinically diagnosed on the basis of urine culture and biological signs in patients.
COM = calcium oxalate monohydrate, COD = calcium oxalate dihydrate, Cys = cystine, OCP = octacalcium phosphate pentahydrate, UA0 = uric acid anhydrous, UA2 = uric acid dihydrate.
ap < 0.01 vs MAP; b p < 10−6 vs MAP.
cp < 0.001 vs CA; d p < 10−6 vs CA.
ep < 10−6 vs metabolic compounds (UA0, UA2, CYS, COM, COD).
fp < 0.001 vs AU0.
gp < 10−4 vs Wk, UA2, COM, COD.
The statistical analysis (Table 1) clearly implicates a new compound as strongly related to UTI, namely Wk. Actually, more than 50% of patients with whitlockite stones had clinically recognized UTI. Such a result is in line with previous publications in which the content of Wk in kidney stones greater than 20%, estimated by FTIR, was related to infection with a high degree (80% of cases) [31]. Such relationship has been found also in a recent publication dedicated to Wk [32].
Considering CA, we also found a relationship between this compound and UTI. More precisely, we have previously noticed a significant association between a high
While MAP, Wk, and apatite have been identified in concretions at the surface of JJ stents, it seems that Wk is quite rare among the chemical phases present. Indeed, in a recent study on the mechanical properties of used JJ stents [40, 41, 42], chemical analysis of the calcifications at the surface of 52 stents shows the presence of different chemicals including ACCP, CA, Br, octacalcium phosphate pentahydrate (OCP), MAP, AmUr, weddellite (calcium oxalate dihydrate or COD), whewellite (calcium oxalate monohydrate or COM), CYS, mucopolysaccharides (MPS), proteins (PRO), and triglycerides (TRG), but no Wk. Such chemical specificity for the JJ stent is confirmed by a statistical study based on 1676 JJ stents characterized at the Crystal Laboratory of Tenon Hospital; only one device had Wk as a major component, and Wk was present in 26 other stents. These observations correspond to 1.6% of cases, while in kidney stones the occurrence of Wk is equivalent to 4.4%. Note that, in the case of MAP, the same percentage is observed for both kidney stones and the JJ stent i.e. 7.2% [37].
2.3. Pathogenesis of IKS
The literature indicates clearly that the pathogenesis of IKS is the consequence of a high urine pH and of the production of
Hydrolysis of urea by urease is a complex process [45]. The first step generates one molecule of ammonia and one of carbamate appears. In aqueous solution, carbamate spontaneously converts into the second ammonia molecule and carbonic acid. Protonation of ammonia (Figure 2) results in a pH increase.
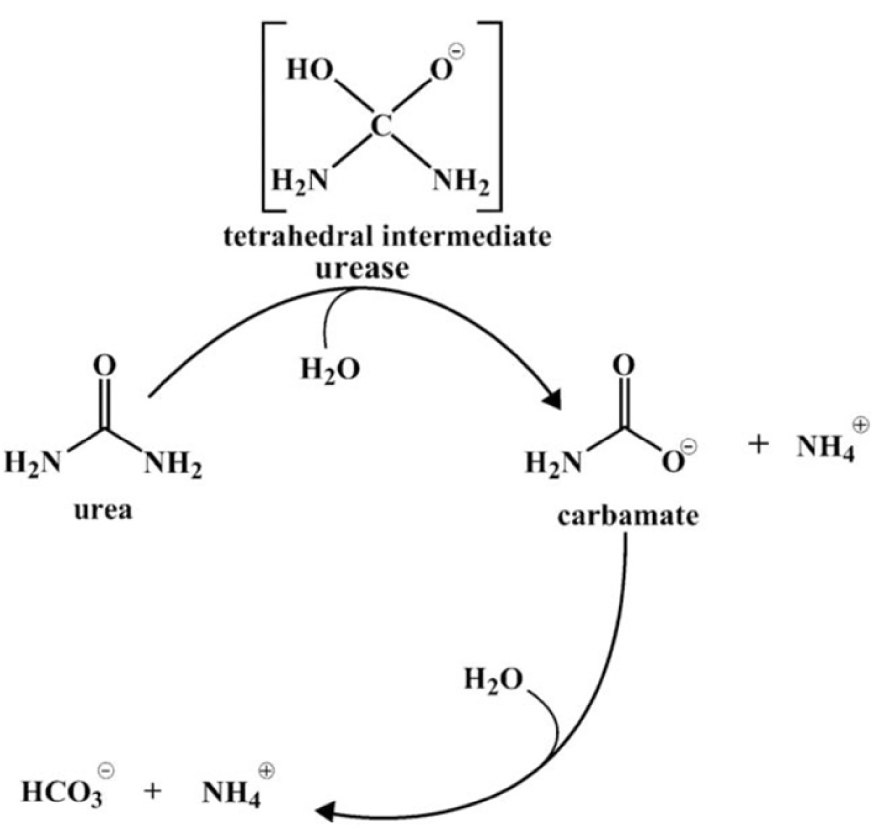
Mechanism of urea hydrolysis (Konieczna et al. [45]).
High urine pH also results in an increased calcium phosphate supersaturation, which facilitates the formation of insoluble salts such as CA and ACCP (Figure 3). However, other changes of urine biochemistry result from ureolysis, such as an increase in
As Figure 3 shows, four chemical phases commonly occur, namely struvite, ACCP, CA and AmUr. Other chemical phases may be present in IKS but an agglomeration of small crystallites trapped in the biofilm produced by bacteria could be an explanation of the presence of these, which may be related to other metabolic disorders as well.
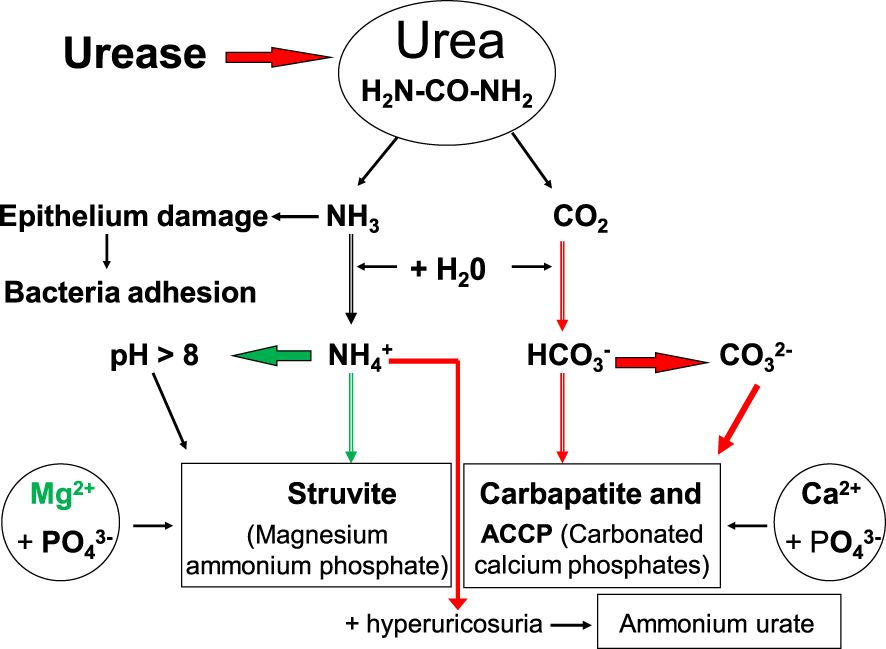
Pathogenesis of infection stones induced by urease-producing bacteria.
From a clinical point of view, one cannot completely exclude the possibility of struvite stones related to conditions other than UTI if urine biochemistry is similar (for other pathological reasons) to that produced by urea-splitting bacteria. To date, no reports of such conditions have appeared in humans. Thus, our discussion of the potential link between UTI and various crystalline phases identified in urinary calculi will omit any unsubstantiated causes of struvite formation.
2.4. Nature of the bacteria related to UTI
As reported in several publications [47, 48, 49, 50, 51], the most common pathogens in UTI are the members of the Enterobacterales order (Gram-negative bacteria found in the gut, namely Escherichia coli, Klebsiella spp., pathogens of the CES group (Citrobacter–Enterobacter–Serratia), members of the Proteae tribe (Proteus–Providencia–Morganella) with a very high proportion of urease-positive strains; other causative agents include Gram-positive cocci (Enterococcus spp., Streptococcus spp., Staphylococcus saprophyticus, Staphylococcus epidermidis and Staphylococcus aureus), non-fermenting Gram-negative bacteria (Pseudomonas spp. and Acinetobacter spp.), atypical microorganisms (Mycoplasma, Ureaplasma species) and yeasts (Candida spp.).
A generally urease negative species is Escherichia coli, among strains of which only about 1% of urease-positive isolates were found. In Table 2 we summarize the main micro-organisms identified in urine samples from 980 patients of our cohort with urinary stones containing any proportion of MAP. Urea-splitting bacteria were most frequent (72.0%).
Microorganisms identified in urine of stone formers with MAP-containing calculi
Bacterial strain | Number of cases | % | Urease |
---|---|---|---|
Proteus mirabilis | 528 | 53.9 | + |
Escherichia coli | 188 | 19.2 | − |
Pseudomonas aeruginosa | 53 | 5.4 | + |
Enterococcus faecalis | 48 | 4.9 | − |
Klebsiella pneumoniae | 42 | 4.3 | + |
Staphylococcus aureus | 29 | 3.0 | + |
Enterobacter cloacae | 25 | 2.6 | − |
Staphylococcus epidermidis | 15 | 1.5 | + |
Corynebaterium urealyticum | 13 | 1.3 | + |
Serratia marcescens | 7 | 0.7 | + |
Citrobacter freundii | 7 | 0.7 | − |
Candida albicans | 6 | 0.6 | − |
Morganella morganii | 5 | 0.5 | + |
Providencia stuartii | 4 | 0.4 | + |
Proteus vulgaris | 4 | 0.4 | + |
Staphylococcus saprophyticus | 4 | 0.4 | + |
Ureaplasma urealyticum | 2 | 0.2 | + |
Total | 980 | 100.0 |
Table 3 shows that the distribution of micro-organism strains in urine from patients who have formed Wk-containing stones is different from that observed for MAP stones.
Occurrence of micro-organisms identified in urine samples from patients who form stones without MAP
Bacterial strain | Patients with Wk-containing stones | Patients with stones without Wk | p vs Wk stones | ||
---|---|---|---|---|---|
Number | % | Number | % | ||
Proteus mirabilis | 20 | 11.8 | 134 | 10.7 | NS |
Escherichia coli | 99 | 58.6 | 632 | 50.2 | NS |
Pseudomonas aeruginosa | 24 | 14.2 | 83 | 6.6 | 0.001 |
Enterococcus faecalis | 7 | 4.1 | 124 | 9.9 | NS |
Klebsiella pneumoniae | 7 | 4.1 | 68 | 5.4 | NS |
Staphylococcus sp. | 5 | 3.0 | 88 | 7.0 | NS |
Enterobacter cloacae | 2 | 1.2 | 24 | 1.9 | NS |
Corynebaterium sp. | 3 | 1.8 | 3 | 0.2 | |
Citrobacter freundii | 2 | 1.2 | 16 | 1.2 | NS |
Candida albicans | — | — | 21 | 1.7 | |
Others | — | — | 65 | 5.2 | — |
Total | 169 | 100.0 | 1258 | 100.0 |
3. Infrared spectroscopy identification of MAP and Wk
Infrared spectroscopy is one of the most common physical techniques used for stone analysis [52, 53, 54, 55, 56, 57, 58, 59, 60]. An infrared spectrum can be considered as the fingerprint of a compound or a mixture of several components in various proportions. However, because minerals, in particular phosphate compounds, exhibit broad peaks and are often present as mixtures in the same stone, it may sometimes be difficult to identify each crystalline phase accurately.
However, for clinical purposes, it is very important to detect the presence of certain components such as MAP or Wk that may be markers for urinary tract infection as the cause of the stone. When MAP is the main component, it is easy to identify as shown in the infrared spectrum of pure struvite in Figure 4. Its main characteristics are a very strong 𝜈3
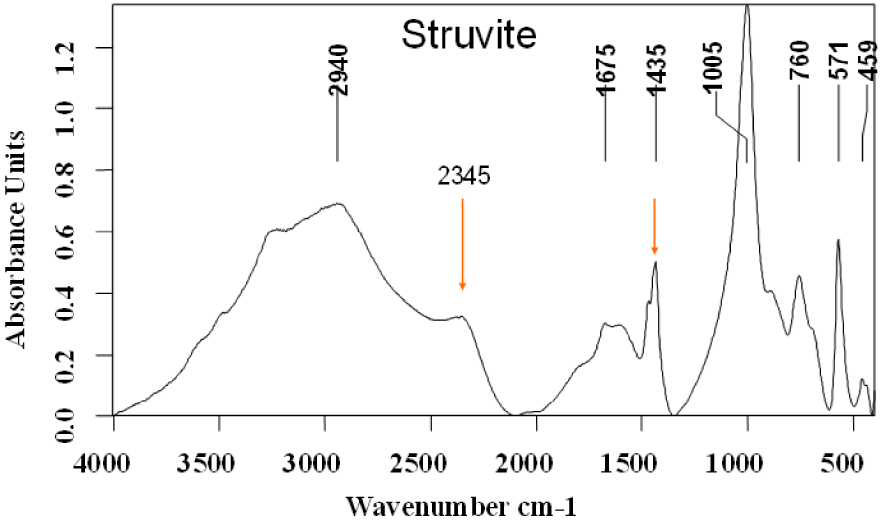
Struvite infrared spectrum.
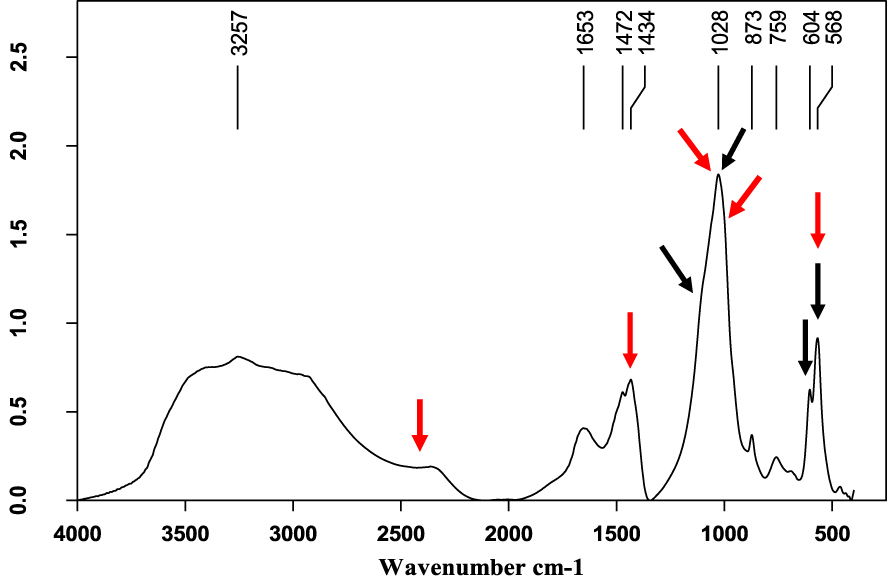
Mixed struvite (red arrows) and calcium phosphate (black arrows) stone.
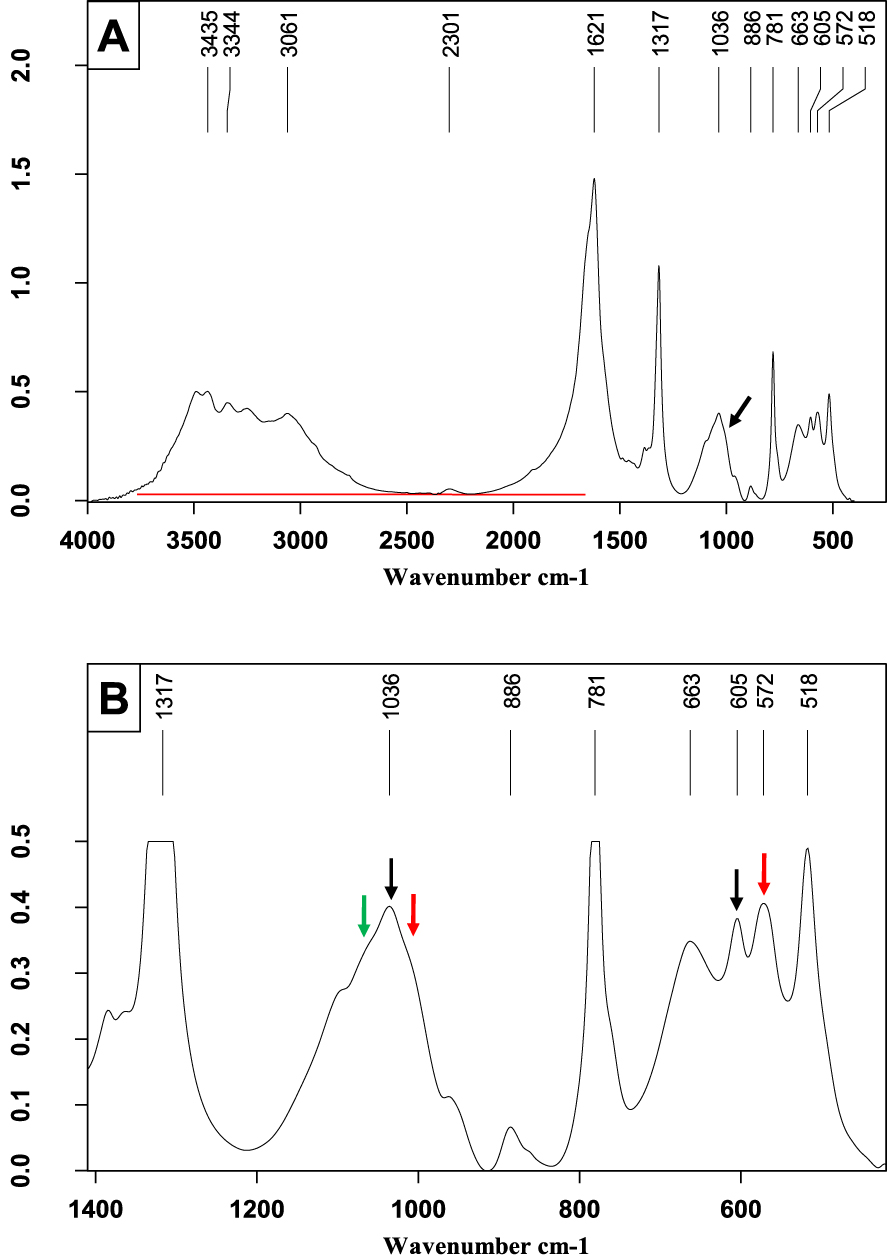
Mixed calcium stone mainly containing calcium oxalate monohydrate with small proportions of ACCP (green arrow), CA (black arrows) and MAP (red arrows). (A) FTIR spectrum on the wavenumber range 4000–400 cm−1. (B) Enlargement of a portion of Figure 6A to better see the shoulders on the broad 𝜈3 phosphate band.
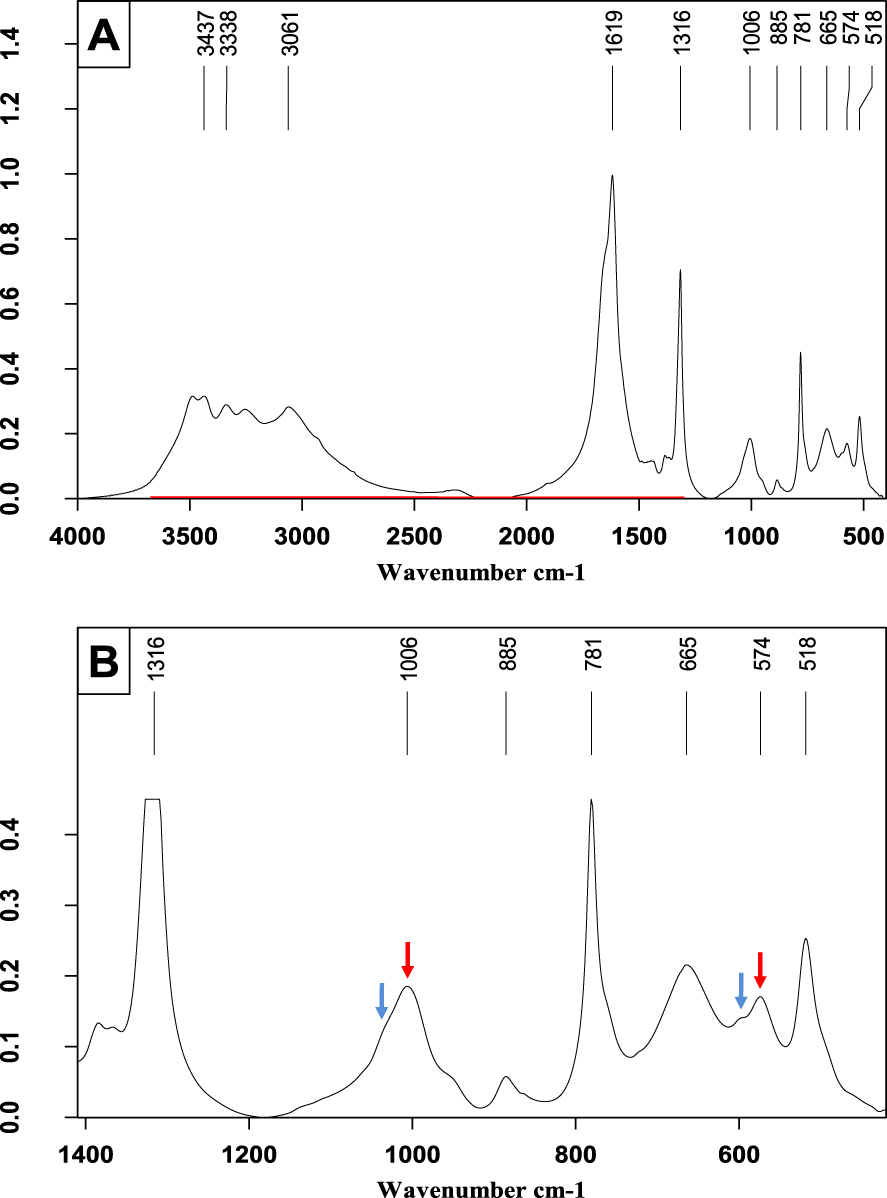
Another mixed stone containing struvite. (A) FTIR spectrum on the wavenumber range 4000–400 cm−1. Note the presence of a plateau between 2500 and 2345 cm−1 indicative of struvite. (B) Enlargement of a portion of Figure 7A to see more easily some details of the phosphate bands. Shoulders at 1035 and 600 cm−1 correspond to CA bands (blue arrows) while peaks at 1006 and 574 cm−1 (red arrows) correspond to struvite. Other peaks indicate the presence of calcium oxalate monohydrate.
When MAP is a minor component in a phosphate mixture, it is of prime importance to detect its infrared bands, mainly the plateau at 2345 cm−1. The 𝜈2 peak of
As shown in Figure 5, in calcium orthophosphate mixtures containing less than 30% MAP, the pseudo-plateau arising at 2345 cm−1 is less intense, the 𝜈3
The same criteria can be useful when MAP is only present as a small proportion of the mixture. For example, the spectrum in Figure 6 mainly shows whewellite (calcium oxalate monohydrate), carbapatite (10%), and struvite (⩽5%). Note that the pseudo-plateau between 2500 and 2345 cm−1 is only very slightly above the baseline at 2100–2150 cm−1. However, other markers of MAP can be used such as the discrete shoulder at 1005 cm−1 (black arrow) and that to the left of the 𝜈4 PO4 peak at 572 cm−1 (red arrow on the expanded spectrum in Figure 6B).
The expanded spectrum (Figure 6B) reveals a third phosphate, namely ACCP, detected by the slight convexity (green arrow) between the 𝜈3
The final spectrum (Figure 7) shows a mainly whewellite composition, with struvite content less than 15%. The pseudo-plateau at 2345 cm−1 is more prominent and the 𝜈3
Nevertheless, the spectrum expansion in Figure 7B reveals shoulders due to the presence of CA (blue arrows) on the left of the struvite peaks. CA accounted for less than 10% of the mixture.
Whitlockite is another compound often related to urinary tract infection. Its infrared spectrum is shown in Figure 8 where a small proportion of proteins may also be detected (black arrows).
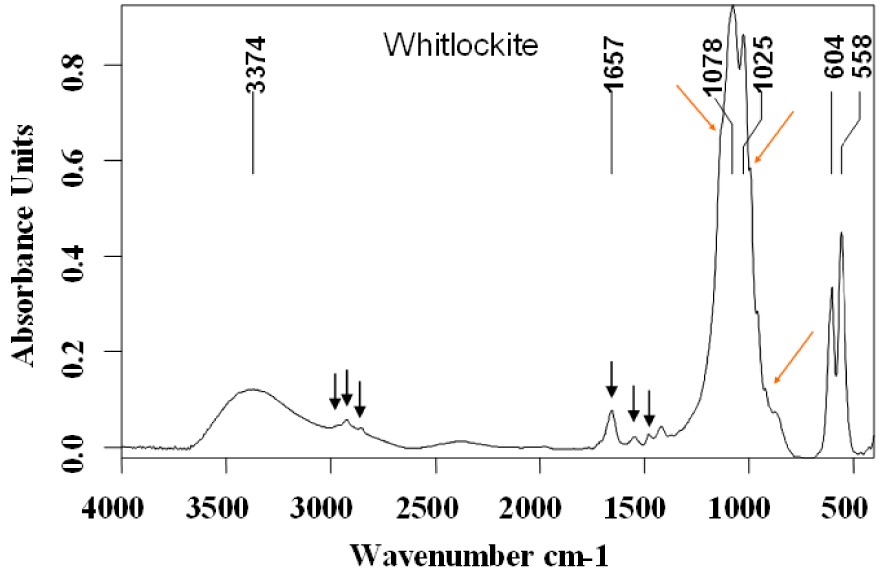
Whitlockite infrared spectrum.
Characteristic vibrations for Wk are observed as a strong band with two maxima at 1078 (𝜈3
As with MAP, Wk is rarely the only phosphate present. It is almost always accompanied by CA. In such cases, the 𝜈4
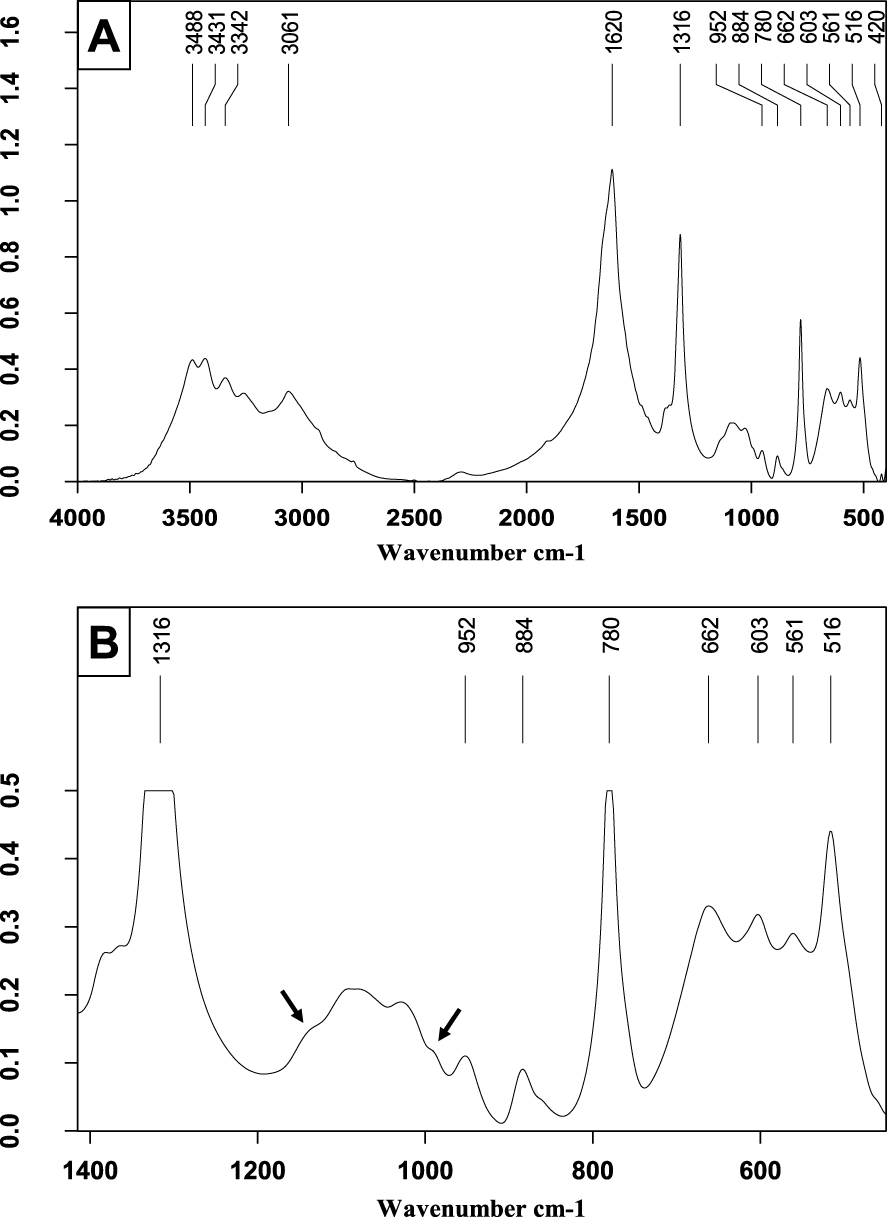
Kidney stone mainly containing whewellite accompanied by a small proportion of whitlockite (about 7–8%) and of carbapatite (less than 5%). (A) FTIR spectrum on the wavenumber range 4000–400 cm−1. (B) Enlargement of a portion of Figure 9A to better see some details of the phosphate bands: shoulders of the whitlockite at 1135 and 991 cm−1 are marked by the black arrows.
In cases where CA is the main component (Figure 10), it is of prime importance to look for other phosphates and particularly Wk. For this purpose, one must detect a shoulder at 990 cm−1 to the right of the 𝜈3 stretching band of CA and another shoulder to its left side at about 1135 cm−1 (see the expanded spectrum). Note that, in addition, the spectrum shows a small proportion of proteins (𝜈3 C–H stretching bands between 2860 and 2960 cm−1).
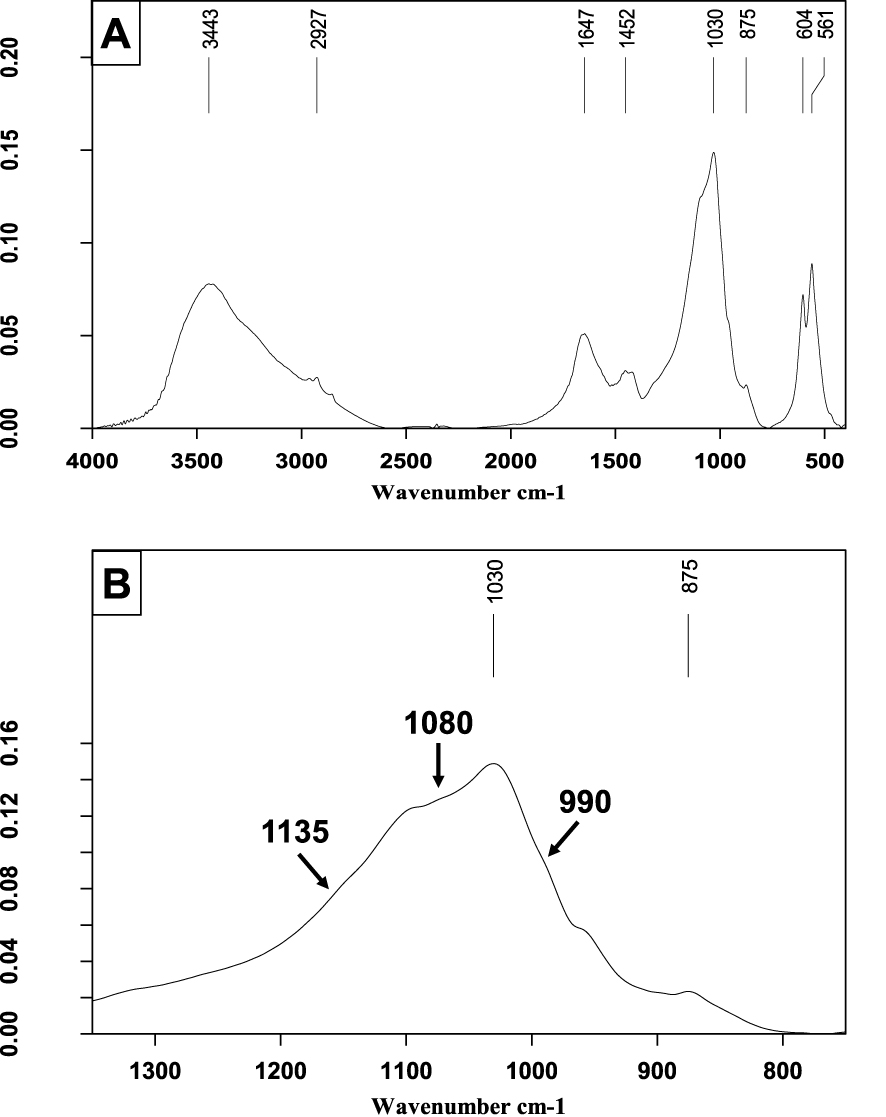
Carbapatite and whitlockite stone. (A) FTIR spectrum on the wavenumber range 4000–400 cm−1. (B) Enlargement of a portion of Figure 10A to see some details of the phosphate bands, especially shoulders of whitlockite at 1135 and 990 cm−1.
4. Physicochemical data regarding IKS
4.1. Crystallographic structure of chemical phases related to infection
4.1.1. Struvite
This compound was named after Heinrich Christoph Gottfried von Struve (1772–1851) of the Russian diplomatic service who was a co-founder of a natural science museum in Hamburg. Struvite (MgNH4PO4⋅6H2O), a white inorganic crystalline mineral, crystallizes in the orthorhombic system with cell dimensions a = 6.941 ± 0.002 Å, b = 6.137 ± 0.002 Å, c = 11.199 ± 0.004 Å. The space group is Pmn21 and there are two molecules in the unit cell [65, 66, 67, 68, 69, 70]. Figure 11A shows the atomic scale structure of struvite and Figure 11B the morphology of the struvite crystal [68]. As we will see, struvite may present very different morphologies.
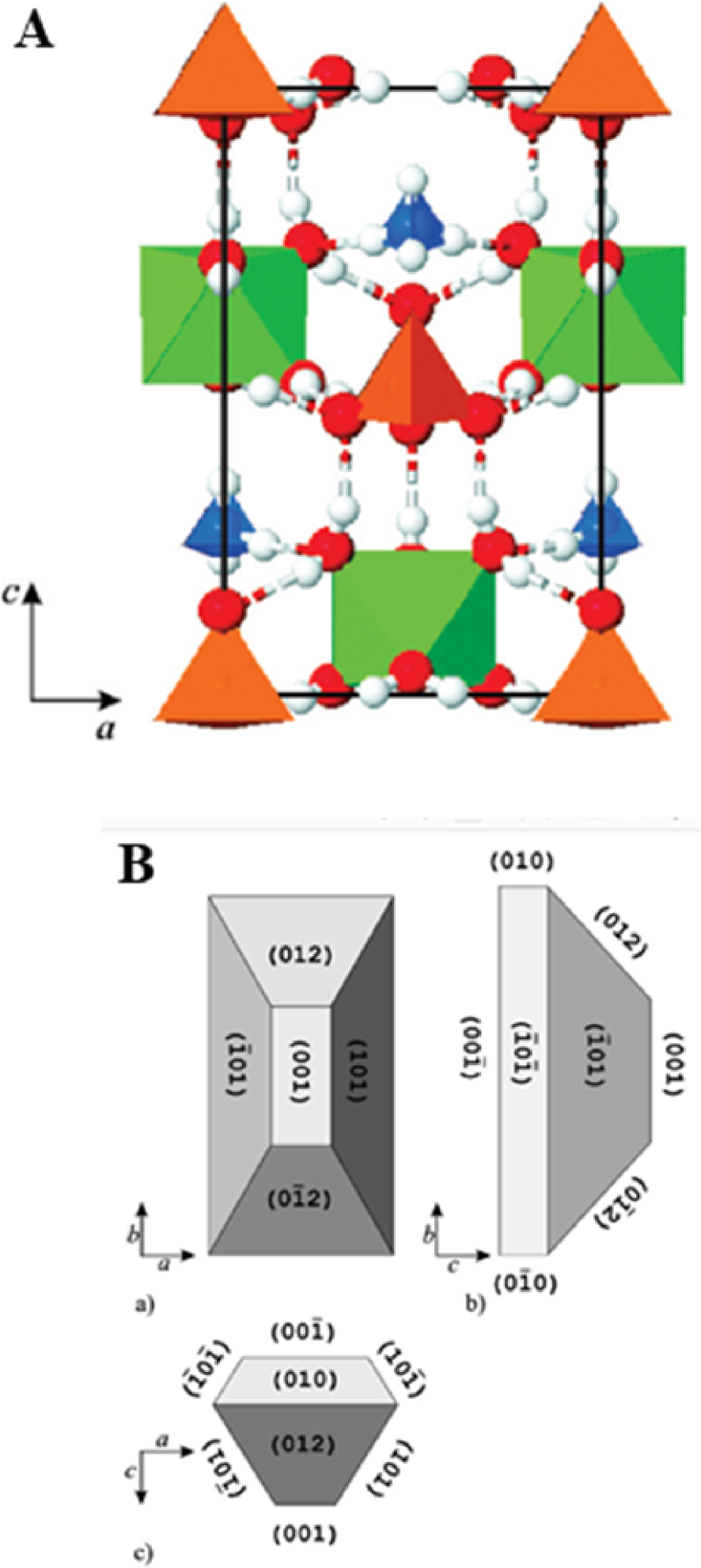
(A) The structure of struvite:
4.1.2. Carbapatite
The mineral’s name is derived from the Greek word απατεí𝜈 (apatein), which means to deceive or to be misleading. The crystallographic structure of calcium phosphate hydroxyapatite (HAP) is well known. The space group is P63/m (Figure 12A), the values for the crystallographic parameters being a = b = 9.41844 Å, c = 6.88374 Å [71, 72, 73, 74, 75, 76, 77].
Biological apatites have some structural specificities. These arise firstly from the stoichiometric formula which in reality is not the canonical Ca10 (PO4)6(OH)2 but in practice Ca10−x+u □x−u (PO4)6−x (CO3)x (OH)2−x+2u □x−2u where squares correspond to vacancies or additional cations and anions while x and u values are as follows: 0 < x < 2 and 0 < u < x. Such complexity takes into account substitution processes [78, 79, 80] as well as Ca2+ and OH− vacancies [81, 82] (Figure 12B). In the case of biological apatites, the diffraction peak (hkl = 002) is sharper than the other peaks, indicating the anisotropy of nanocrystals (needle and/or platelet-like morphology) [83]. Note that a structured hydrated layer exists at the surface of biological apatite which serves as an exchange area with the biological environment (Figure 12C) [84, 85, 86, 87].
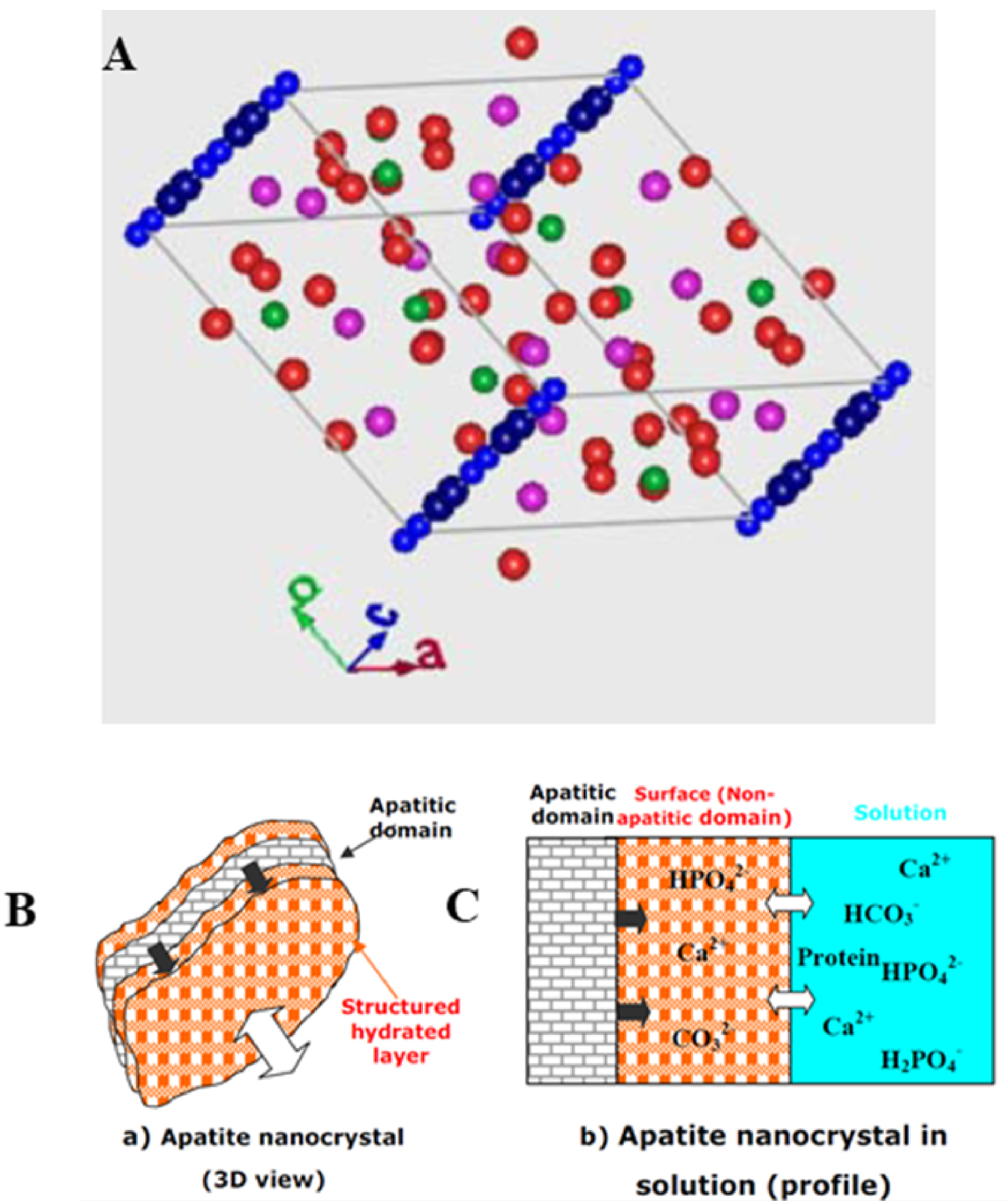
(A) Spatial distribution of different atoms in HAP. Hydrogen and oxygen atoms of the hydroxyl groups represented in blue are located on the c-axis. (B) and (C) Schematic model of the surface hydrated layer of poorly crystalline apatite nanocrystals (from Refs. [84, 85]).
4.1.3. Whitlockite
Whitlockite was named after Herbert Percy Whitlock (1868–1948), a curator of minerals at the American Museum of Natural History in New York (USA). Wk is a complex material which involves cation substitutions, cation vacancies, and protonation of phosphate groups [88, 89, 90]. Using quantum chemical structural optimization by Density Functional Theory (DFT) calculations, Debroise et al. [90] were able to quantify the ability of Wk to hold these substitutions and vacancies in preferential sites. The impact of Ca2+/Mg2+ substitutions on X-ray diffraction (XRD), IR, and Raman characteristics fell within the scope of DFT and compared with experiment. In particular, the crystallographic positions of the vacancy and optimal Ca+2/Mg2+ substitution sites were identified (Figure 13). Mg2+ concentration could be quantified from the variation in length of the unit cell parameters. Also, they show clearly that Raman spectroscopy is very sensitive to the Mg2+ substitutions and to the presence of impurities such as Fe2+. The theoretical IR spectra enabled unequivocal assignment of observed spectral bands to specific vibrations. As with Raman, IR spectra were found to be very sensitive to the presence of defects, and the Mg2+ concentration.
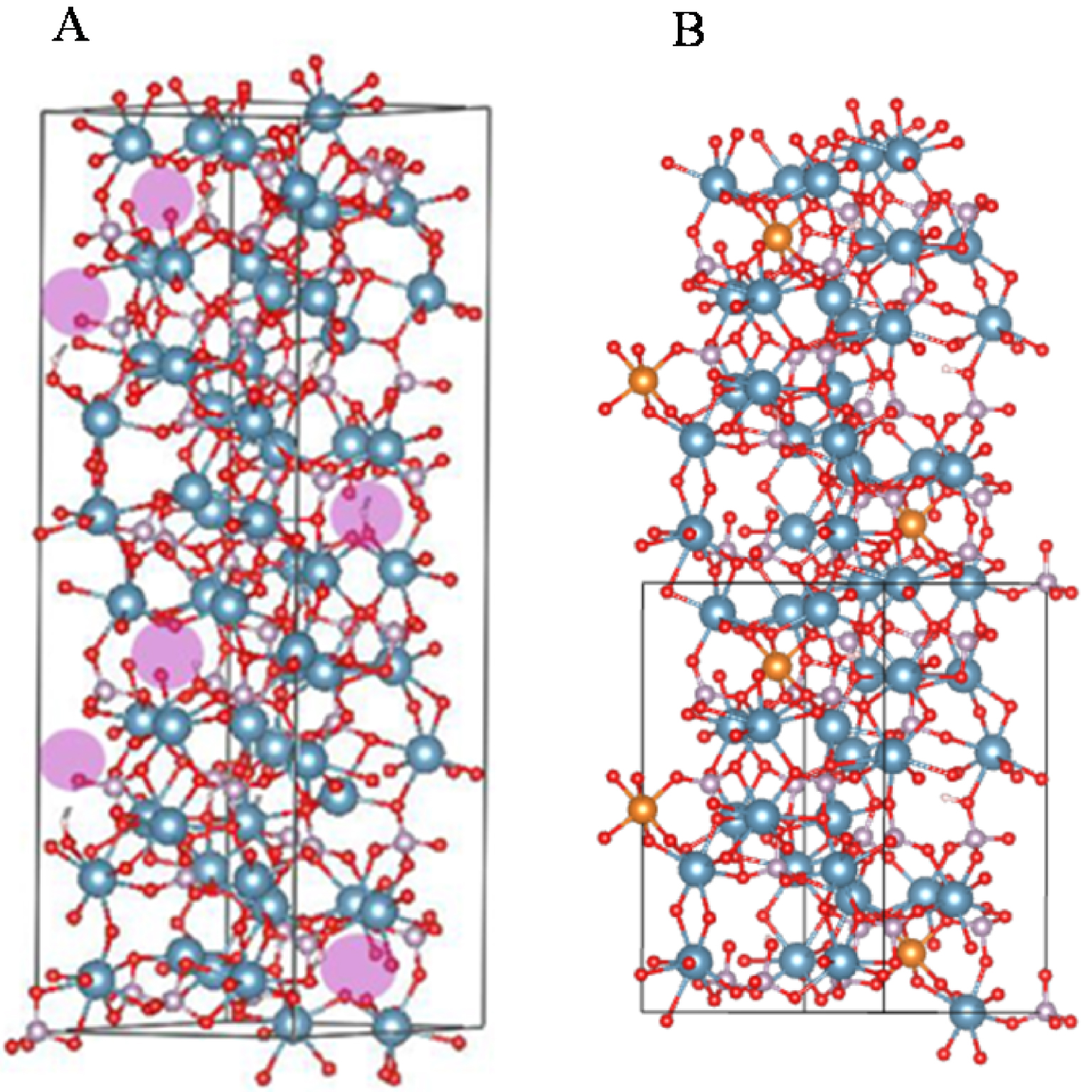
(A) Whitlockite unit cell showing vacancies indicated by purple spheres, which correspond to the crystallographic Ca2+ site number 4. (B) Whitlockite unit cell showing the half unit cell with 3Mg2+ substitutions on the Ca2+ sites. Crystallographic site 5 was found to be the most favourable energetically for substitution by Mg2+. Ca: blue, Mg: gold, P: grey, O: red and H: white (from Debroise et al. [90]).
4.2. Synthesis of chemical phases related to IKS
In this section, we will not describe the synthesis of calcium phosphate apatite as its presence in bone has resulted in a considerable literature (see for example [91, 92, 93, 94, 95, 96, 97]).
4.2.1. The case of struvite
Several papers, arising in two very different research fields, wastewater treatments, and medicine, describe the synthesis of MAP [98, 99, 100, 101, 102, 103, 104, 105, 106, 107, 108, 109, 110, 111]. Different precursors have been used in in vitro experiments. For example, introduction of Mg2+ cations can be performed by addition of MgSO4, MgCl2⋅6H2O, C4H6MgO4 to the synthesis solution. In the case of Ca2+ cations, CaCl2⋅2H2O is usually used. Some of these synthetic struvite crystals, such as the one published by Manzoor et al. [106], have been obtained in the presence of creatine.
In urine, crystallization of struvite is highly dependent on pH and relative concentrations of magnesium, phosphate and ammonium as shown in Figure 14.
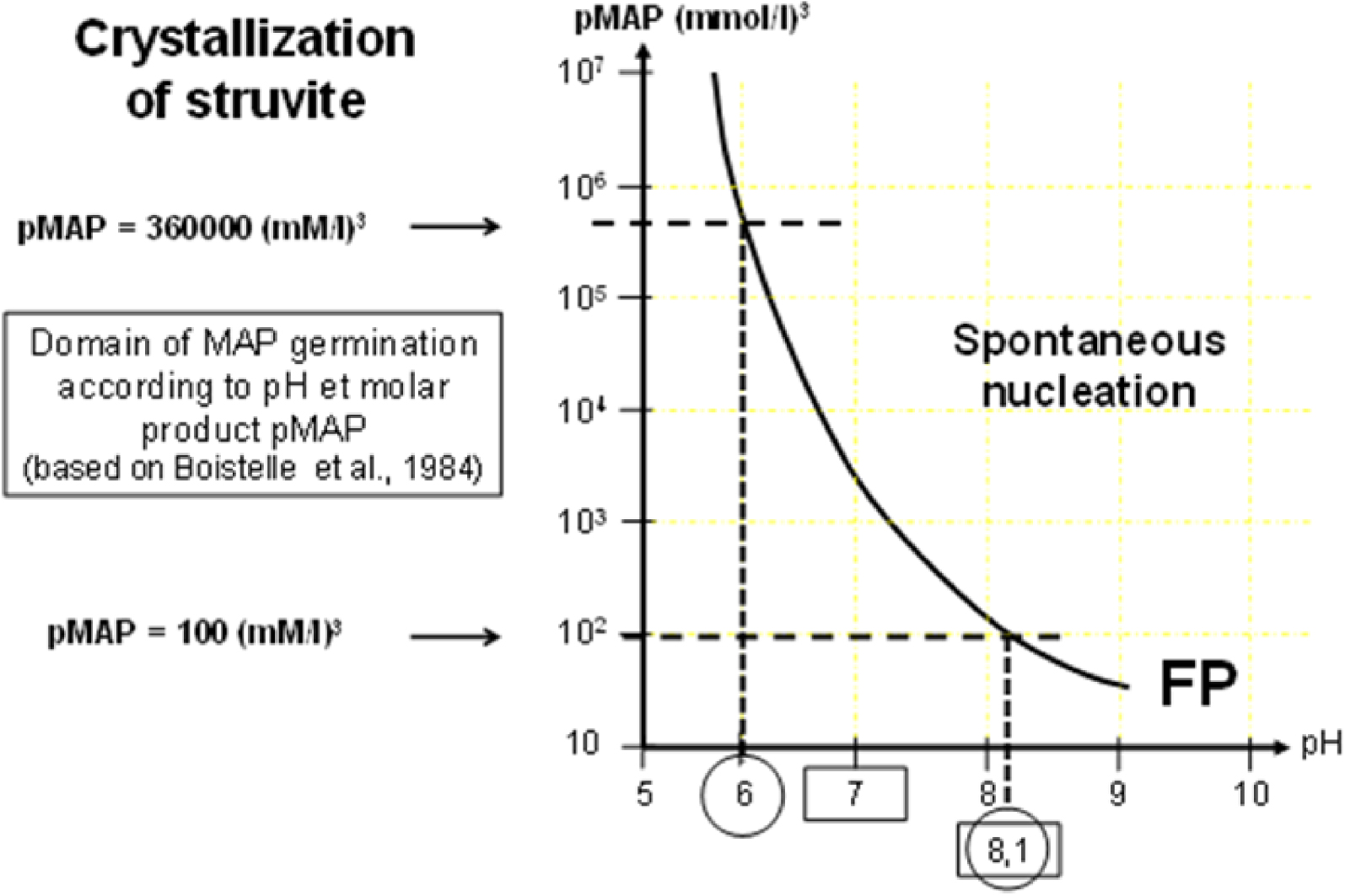
Crystallization of struvite is highly dependent on urine pH. FP is the formation product of MAP, i.e., the limit of pMAP above which MAP crystals spontaneously form in urine.
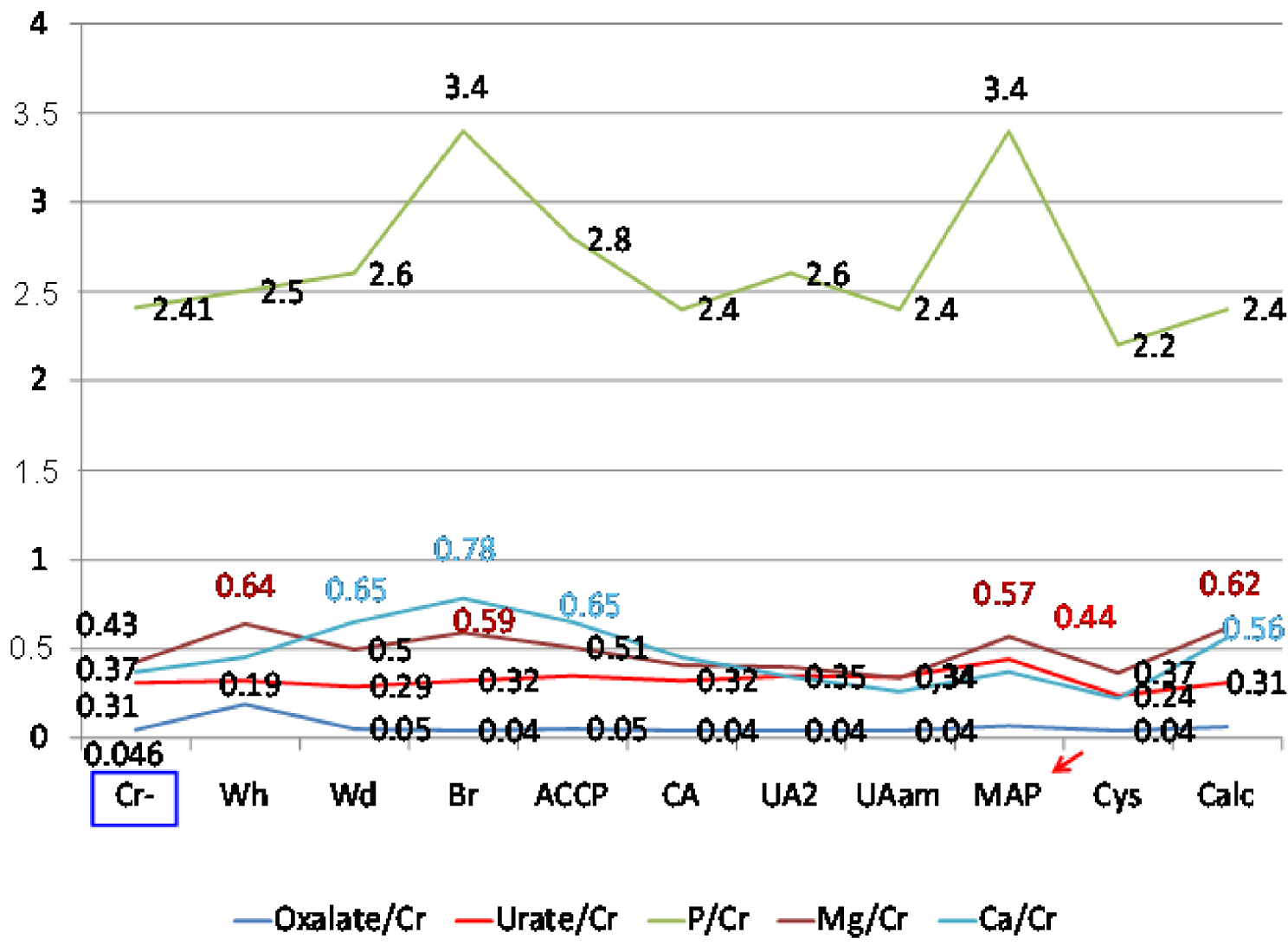
On the y axis, are shown the mean values of oxalate to creatinine ratio (), urate to creatinine ratio (
), phosphate to creatinine ratio (
), magnesium to creatinine ratio (
) and calcium to creatinine ratio (
) for the different crystalline phases described below. Cr− = absence of crystals. Crystalline phases: Wh = whewellite; Wd = weddellite; Br = brushite; ACCP = amorphous carbonated calcium phosphate; CA = carbapatite; UA2 = uric acid dihydrate; UAam = uric acid amorphous; MAP = struvite; Cys = cystine; Calc = calcite. Masquer
On the y axis, are shown the mean values of oxalate to creatinine ratio (), urate to creatinine ratio (
), phosphate to creatinine ratio (
Lire la suite
For a similar magnesium ammonium phosphate molar product (pMAP), the relative concentrations of ions involved in pMAP can be very different as shown in Table 4, explaining the fact that bacterial urease activity is not the unique condition for struvite crystallization and that the urine biochemistry of the patient can also be involved (Figure 15). Nevertheless, as shown in Figure 14, urine pH is a very strong determinant for MAP crystallization. Below pH 6.5, the concentrations of magnesium, ammonium, and phosphate, required for MAP formation are too high to correspond to any physiological or pathological condition; this is why MAP in urinary concretions is commonly considered a marker of alkaline urine related to urinary tract infection by urea-splitting bacteria.
Formation product (FP) for MAP (pMAP) at different urinary pH and ion concentrations (adapted from Boistelle et al. 1984 [110])
Ion concentration (mmol/L) | Formation product (FP) of pMAP (μmol/L3) | pH at FP | ||
---|---|---|---|---|
Mg | PO4 | NH4 | ||
1 | 5 | 10 | 50 | 8.7 |
1 | 10 | 15 | 150 | 8.0 |
1 | 10 | 50 | 500 | 7.45 |
2 | 20 | 20 | 800 | 7.3 |
3 | 20 | 30 | 1800 | 6.95 |
2 | 10 | 100 | 2000 | 7.0 |
2 | 100 | 10 | 2000 | 7.0 |
4 | 30 | 30 | 3600 | 6.8 |
6 | 30 | 40 | 7200 | 6.75 |
3 | 10 | 300 | 9000 | 6.7 |
7 | 30 | 50 | 10,500 | 6.65 |
7 | 40 | 80 | 22,400 | 6.5 |
7 | 50 | 100 | 35,000 | 6.4 |
7 | 100 | 200 | 140,000 | 6.15 |
8 | 150 | 300 | 360,000 | 6.0 |
As shown in Table 4, the FP for MAP required for crystallization is very high for pH < 6.8 and rarely encountered in urine. In cases of UTI by urea-splitting bacteria, the increase of urine pH by production of NH3 from urea strongly reduces the pMAP needed for crystallization, mainly encountered in UTI by micro-organisms with urease. However, metabolic conditions which allow MAP crystallization without infection should not be ruled out. This might explain the discrepancy between the occurrence of clinically recognized UTI reported by physicians and the presence of MAP in stones although to date no metabolic condition has been clearly identified as a cause of MAP stones without UTI in humans.
However, such conditions have occasionally been reported in animals [111]. Another explanation is related to the time elapsed between stone formation and stone removal. In the absence of clinically recorded UTI with stones that contain MAP, we noted that MAP was mainly located in the nucleus of the stone (89.8% of cases), which could be a marker for a transient episode of UTI.
As shown in Figure 15, the urinary excretion of solutes markedly reflects the risk of crystallization of a given species. For example, in the case of MAP and brushite crystals, and to a lesser extent for ACCP, the excretion of phosphate ions is significantly higher than for other crystalline species, irrespective other parameters such as urine pH.
4.2.2. The case of whitlockite
Only a few publications report the synthesis of Wk [112, 113, 114, 115, 116, 117], among them note the work of Jang et al. introducing a new synthetic route to pure Wk nanocrystals precipitated under Mg2+-rich and physiological conditions [113]. Qi et al. [114] generated Wk microspheres which displayed high biocompatibility and an excellent ability to promote adhesion and spreading of MC-3T3 osteoblasts using a novel microwave-assisted method. Also, hydrothermal growth of Wk on a β-TCP surface to enhance osteogenesis has been investigated by Guo et al. [115]. Finally, Wk nanoparticles can also be prepared by precipitation using calcium hydroxide and magnesium hydroxide [116].
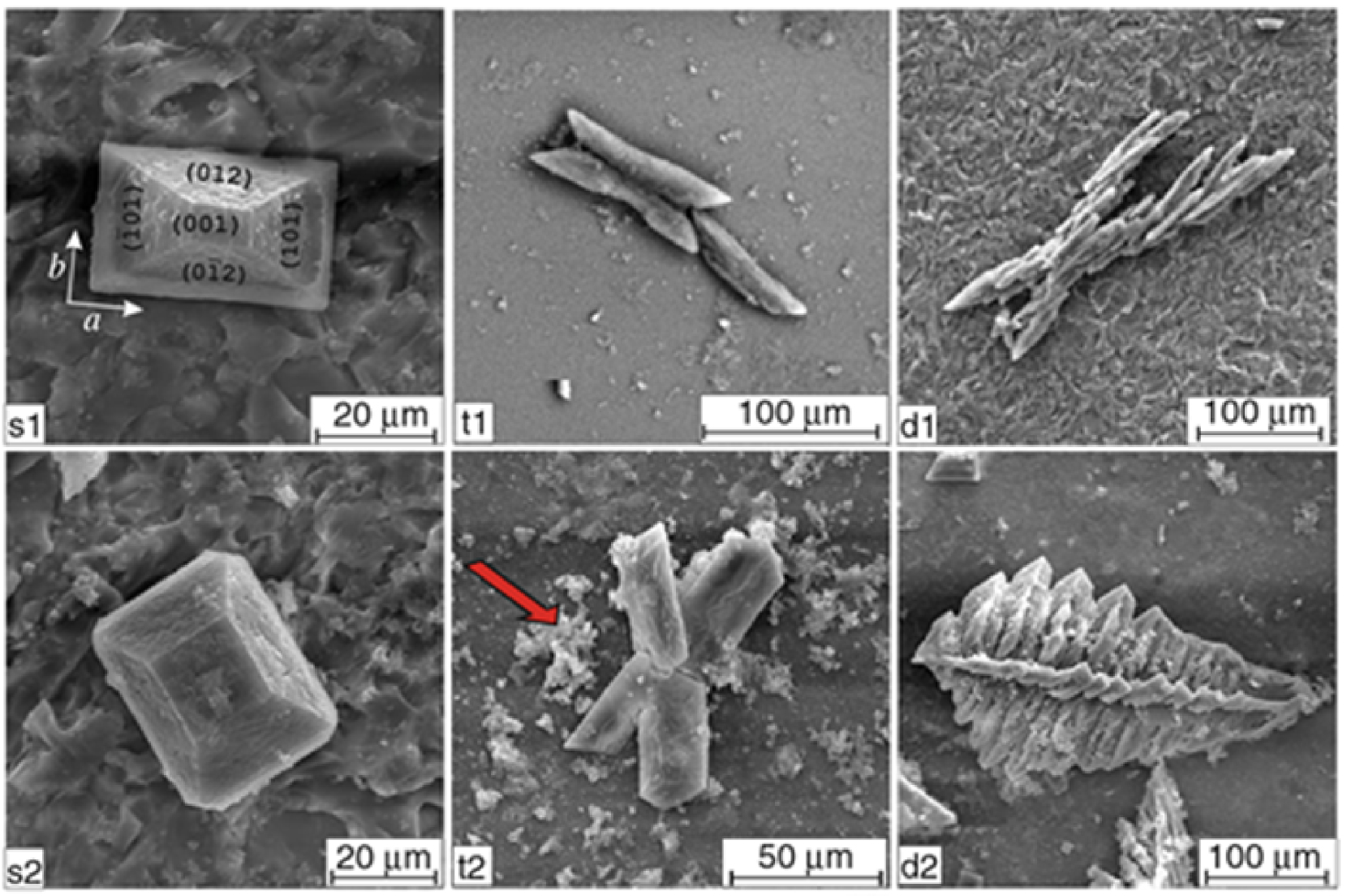
SEM micrographs illustrating the dependence of morphology and habits of struvite on pH of artificial urine; left column: single hemimorphic crystals obtained at pH range 7.2–9.0; middle column: contact (t1) and penetration (t2) twins obtained at pH range 9.0–9.5; right column: X-shaped (d1) and fern-leaf (d2) dendritic crystals obtained at the highest pH values (pH 9.5); arrow indicates CA precipitation (from Prywer et al. [119]).
Recently, Wang et al. [117] have developed an interesting synthesis of Wk nanocrystals using a tri-solvent system for the solid–liquid–solution process, which offers the opportunity to generate Wk nanocrystals with tuneable size, morphology (nanoplates, nanospheres), and surface properties (hydrophobic, hydrophilic), impossible to achieve using the traditional precipitation method.
Regarding the role of pH in the synthesis of Wk, it is worth underlining that, as noted by Jang et al. [113], Wk had greater stability than HAP in acidic conditions below pH 4.2. These authors also confirmed that calcium phosphate apatite can directly transform into Wk under appropriate acidic pH conditions with a sufficient Mg2+ cations content in the medium.It is notable that, in urinary stones, Wk has occurred mixed with MAP in 11.3% of cases and that a mixture of WK and MAP was found in the same layers of more than 100 stones, suggesting that acidic conditions are not required for Wk formation under biological conditions.
4.3. Crystallite morphology of chemical phases related to IKS
4.3.1. Morphology of MAP crystallites
Various publications have reported the morphology of MAP crystallites [118, 119]. Prywer et al. [119] have used scanning electron microscopy to correlate crystal morphology with the pH of artificial urine (Figure 16).
As shown by Daudon and Frochot [120], MAP is highly dependent on urinary pH and may occur in a range between 6.8 and 9.0 (Figure 17).
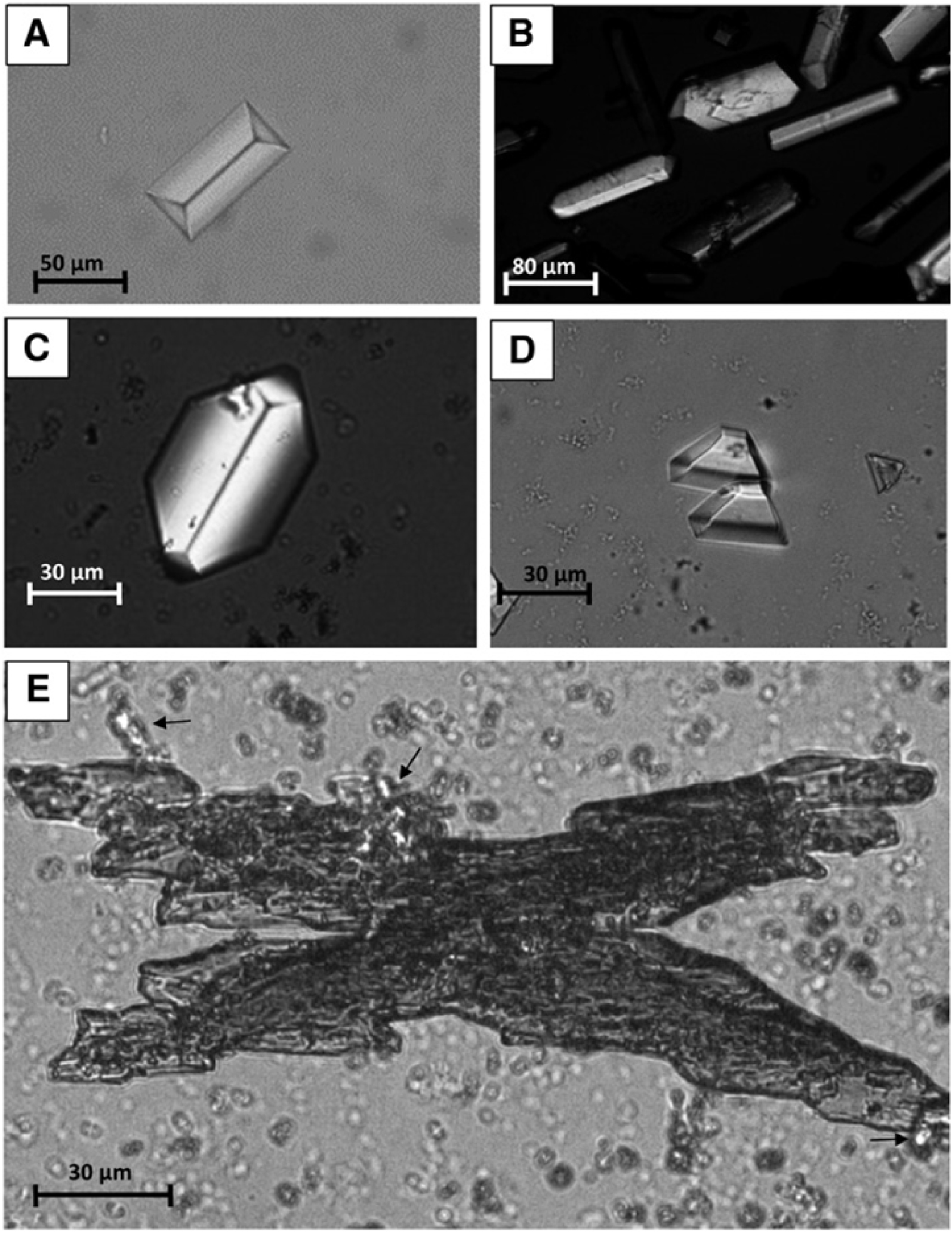
MAP crystals as seen under polarized light (from Daudon and Frochot, 2015 [120]). (A) A coffin-shaped crystal of MAP. (B) Rod-shaped and coffin-shaped crystals of MAP. (C) A hexagonal crystal of MAP and small agglomerates of amorphous carbonated calcium phosphate grains. (D) Trapezoidal crystals of MAP. (E) A large X-shaped dendritic crystal of MAP with birefringent small aggregates of ammonium hydrogen urate crystals (black arrows).
At this point, it is worth underlining those bacteria are able to affect and modify the dimensions or the morphology of MAP crystals [118, 119, 120, 121, 122, 123]. For example, the results of Sun et al. [123] show that MAP particles produced in the presence of bacteria are larger than in the absence. It is possible that bacterial biomolecules may act as templates to induce the nucleation, growth, and aggregation of struvite crystals.
4.3.2. Morphology of CA and Wk crystallites
Several investigators have reported the presence of bacterial imprints in renal stones using transmission and scanning electron microscopy [124, 125, 126]. Indeed, this approach may help identify bacteria that may contribute to stone formation, even though urine culture results might be negative.
More specifically, it was reported that in IKS bacterial imprints are only observed on areas composed of CA and not on MAP crystals [127], as has also been observed in IKS containing Wk (Figure 18). In a contribution to this special issue we have shown that Wk crystallites display a specific morphology [32].
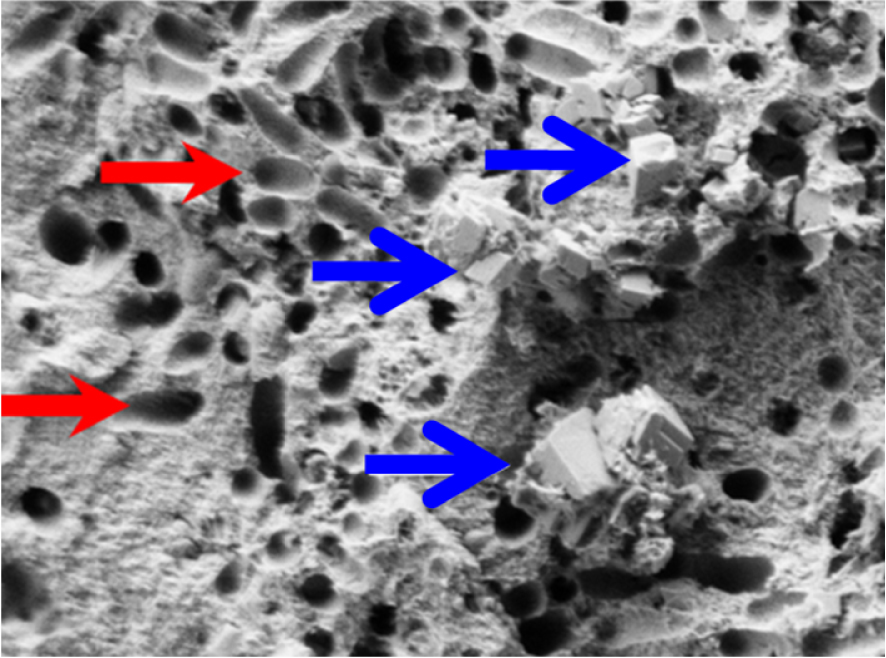
SEM image showing bacterial imprints (red arrows) in a stone made of whitlockite (blue arrows) mixed with carbapatite.
5. Conclusions and perspectives
The comprehensive set of clinical and physicochemical data presented in this contribution shows clearly that IKS display a complex chemistry which is clearly not limited to struvite. For the clinician, such chemical complexity calls for a precise analysis of different infrared spectra related to the core and the surface of a kidney stone. With the increase of bacterial antibiotic resistance and the paucity of new antibiotics (as underlined by the World Health Organization),1 it is quite clear that IKS constitute a major topic of interest for the medical community. It is thus of primary importance to accurately detect the presence of MAP and Wk in urinary stones as well as to precisely measure the carbonation rate of calcium phosphate apatite by FTIR spectroscopy.
Conflicts of interest
Authors have no conflict of interest to declare.