1. Introduction
Since its discovery in the 50s, the field of Olefin Metathesis (OM) has been growing exponentially in terms of applications [1, 2, 3, 4, 5]. Early industrial applications were found in petrochemistry, where the use of heterogeneous molybdenum or tungsten catalysts has proved effective in the production of basic chemical intermediates [6]. Through the years, metathesis reaction (MR) greatly enlarged its cohort of applications, dramatically influencing the current way to think the chemical synthesis (especially true for fine chemicals), and is an invaluable tool in different industrial sectors, ranging from oleochemistry, materials, pharmaceutical, agrochemical and perfume industry [7]. Decades of efforts were finally recognized by the international scientific community with the Nobel Prize awarded in 2005 to the pioneering work of Grubbs, Schrock, and Chauvin [5].
With the isolation of the first stable alkylidene complexes based on tantalum, molybdenum, and tungsten by Schrock in the 80s, the metathesis catalysts are constantly evolving structurally, with a strong progress in the 90s following the development of the first Grubbs catalysts based on ruthenium, which are air-stable and tolerant toward many organic functionalities. The emergence of stable and isolable N-heterocyclic carbene (NHC) ligands (e.g., SIMes, IMes, SIPr) has greatly contributed to improving the stability and activity of Grubbs-type complexes, based on the work of Hermann, Grubbs, and Nolan groups [8]. Another milestone in the structural improvement of metal complexes, resulted in the introduction of styrenyl ether carbenes by Hoveyda et al. [9], offering an increased stability of the precatalyst, characterized by a reservoir effect that greatly improved the catalytic efficiency upon activation [10]. Later on, many other research groups, including the Blechert, Grela, Fürstner, Plénio, and Mauduit groups, contributed to the development of new catalytic species targeting both stability and efficiency [8]. A plethora of ruthenium-based complexes have been developed, constantly pushing the limits of the applications. Many of these catalysts are commercially available and some of them are now produced on multikilogram scale, with intense application in the production of active pharmaceutical ingredients (APIs), notably for the preparation of hepatitis C antivirals (e.g., Ciluprevir), kinase inhibitors (e.g., Pacritinib), and Cathepsin K inhibitors (e.g., Relacatib) (Figure 1) [11, 12]. Marketed since 2014 by Janssen Pharmaceutical, Simeprevir (hepatitis C virus inhibitor) represents the first API industrially produced by a metathesis route, demonstrating the economic viability of this homogeneous transformation [13, 14].
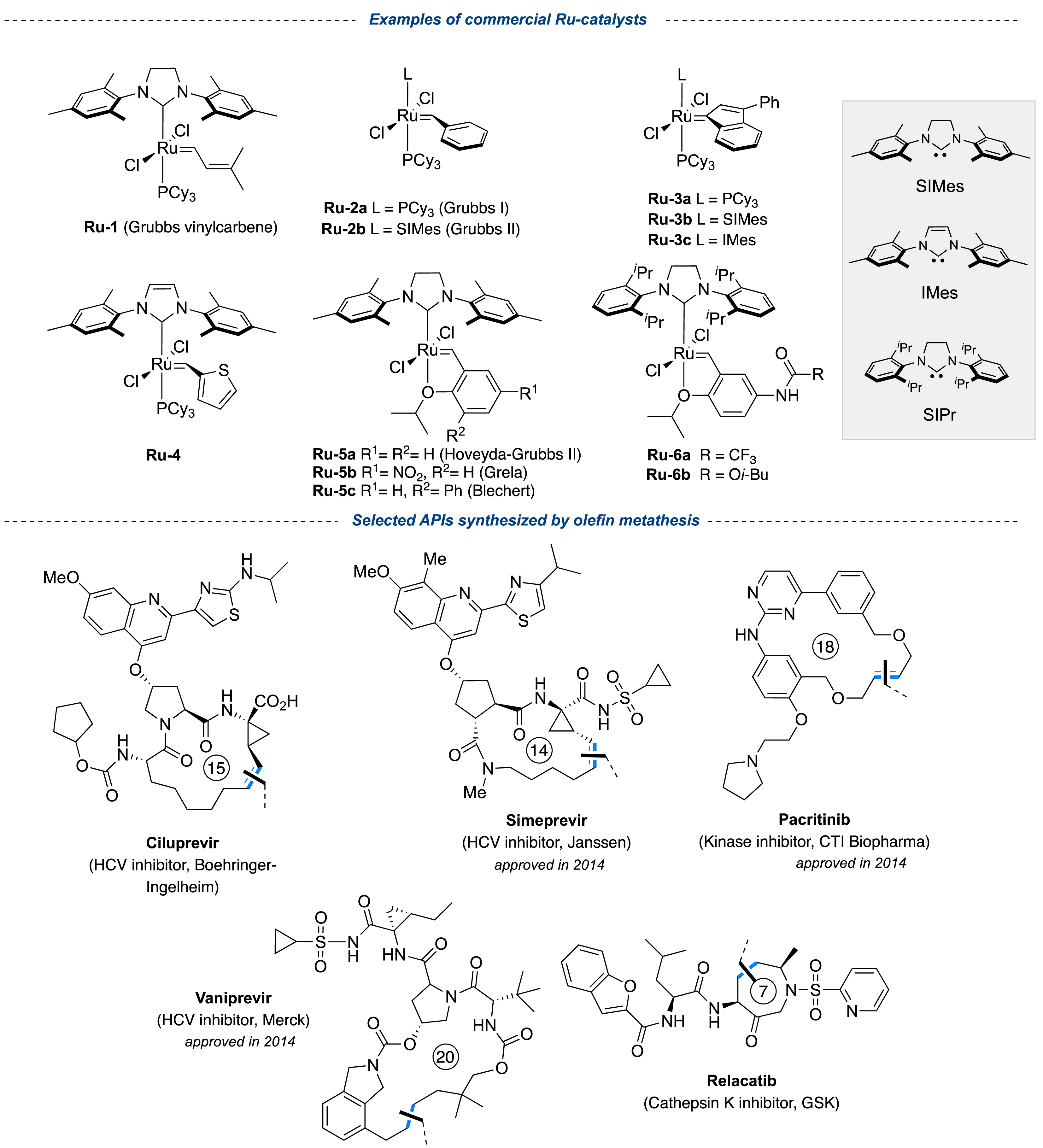
Examples of commercial ruthenium catalysts (available in kg), frequently used in olefin metathesis and selected APIs synthesized in kg scale.
Although the pharmaceutical industry has already integrated the olefin metathesis into manufacturing processes, the application of this technology to the production of commodities from biomass still remains underexploited [11, 15]. Indeed, as Nature is unquestionably a source of olefins, among whose terpene derivatives or triglycerides found in vegetable oils [16], the transformation of biomass by a metathesis reaction is a promising approach to replace synthetic petroleum derivatives in obtaining surfactants, lubricants, monomers for the manufacture of materials, fragrances, or even insect pheromones (Figure 2) [17, 18].
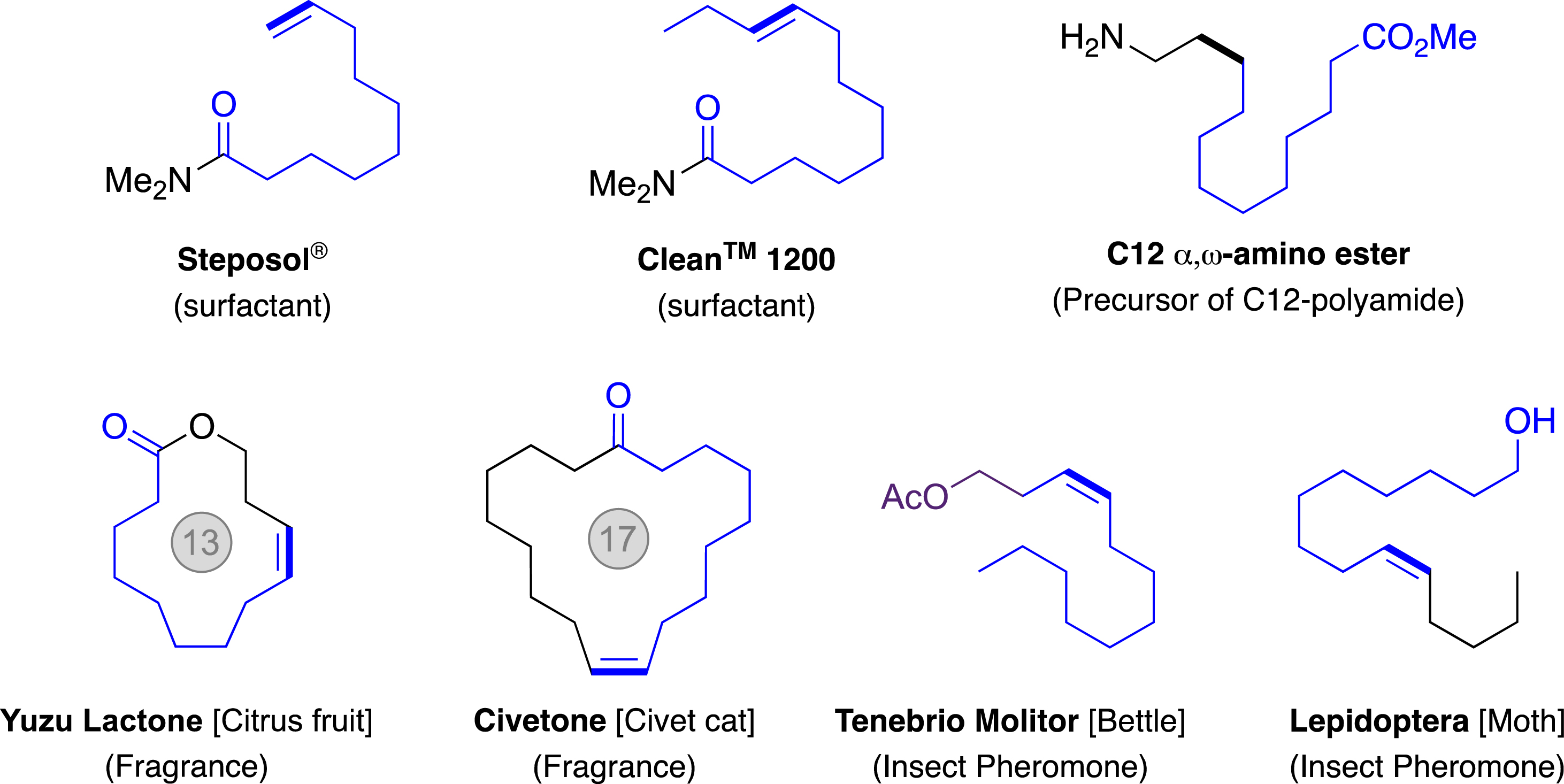
Examples of molecules of interest for which at least one synthesis step is accessible by biomass transformation via olefin metathesis (in bold: bond formed by metathesis; in blue: carbon chains from biomass).
Nevertheless, olefin metathesis should ideally involve low-cost metal catalysts with excellent efficiency (at ppm level) in terms of turnover numbers (TON). Although some metal catalysts are available on a multi-kg scale, the fluctuating market value of transition metals has to be taken into account and the frequently excessive cost still remains one of the weak points of this method [12, 19]. Additionally, the introduction of more complex ligands may greatly impact the overall costs as well. The robustness and stability of metal complexes toward the naturally occurring impurities in raw materials, especially when these are of vegetable origin, is also a selection criterion. The selectivity also plays a pivotal role for the right choice of the catalyst to form the targeted metathesis product only, excluding dimers, oligomers, or positional isomers (resulting from an isomerization of the double bond, see Figure 9). Low cost, robustness and selectivity are therefore the catalyst requirements [20].
At present, the design of ruthenium-based catalysts has evolved dramatically, leading to the synthesis of organometallic species adaptable to the most common chemical challenges [8], including the transformation of biomass [16, 17]. Listing all of them would be extremely reductive, but some particular developments are worth mentioning. With the Grubbs and Bertrand groups’ pioneering work, it was found that the replacement of the classic NHCs (see Figure 1) with cyclic(alkyl)(amino)carbenes (CAAC) [21], successfully impacted on the stability of the methylidene intermediate, thanks to peculiar steric and electronic properties of this carbene (Figure 3) [22]. As such, the ethenolysis, a cross-metathesis (CM) reaction between methyl oleate and ethylene, could be successfully carried out, even using ppm or ppb of catalyst loading, allowing the production of highly desirable biosourced building blocks with very high TONs (up to 2.6 million) (Figure 5) [22, 23, 24, 25, 26, 27, 28]. Of note, these impressive TONs required the use of ultra-highly pure raw materials, which severely impacts the production costs and is therefore not in line with market expectations (Figure 3).
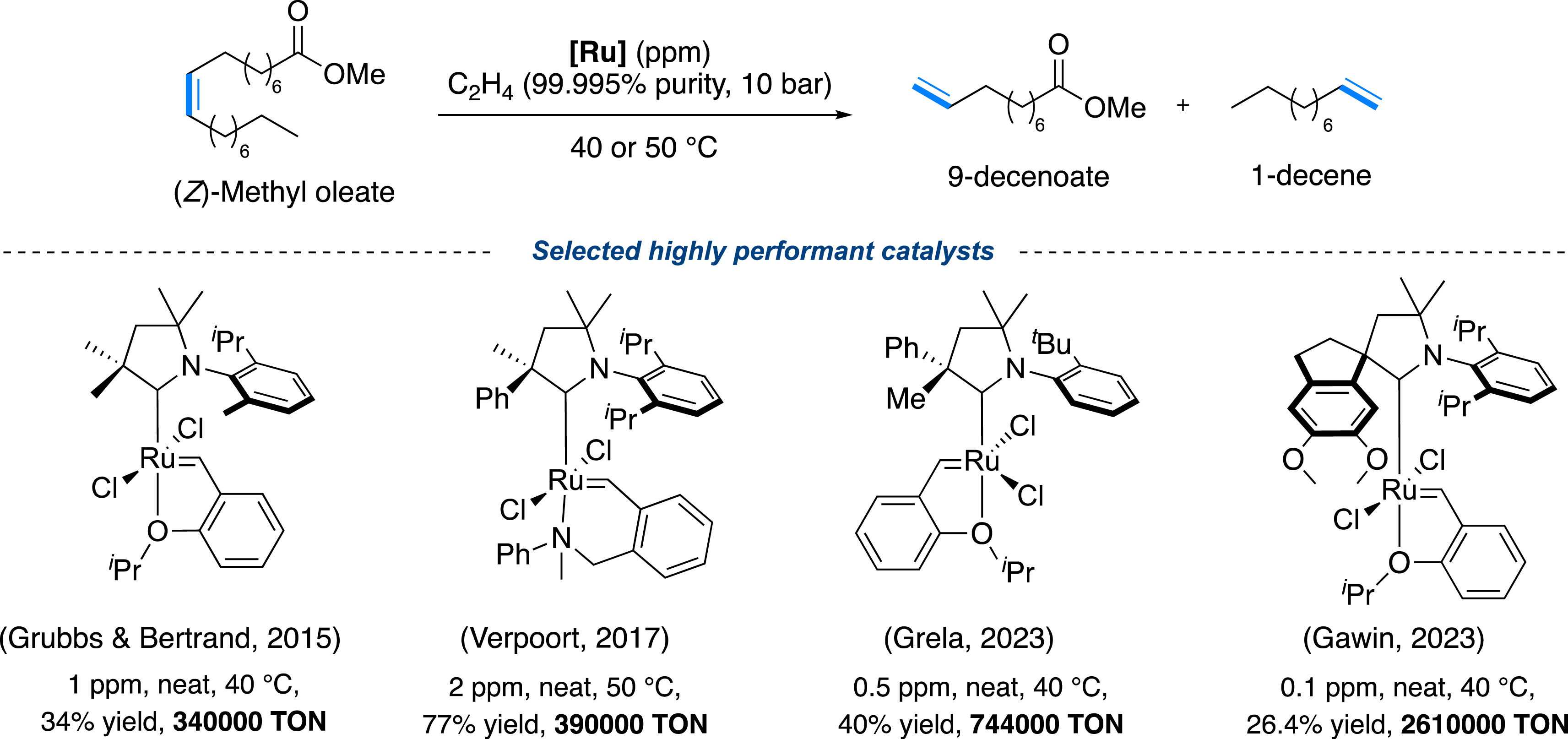
Ethenolysis of (Z)-methyl oleate catalyzed by highly performant CAAC–Ru complexes.
Despite the numerous process patents filed over the last decade (e.g., by Elevance—Wilmar/Cargill, IFPEN, Croda, Versalis, and Arkema, to name a few), there are only limited number of industrial-scale operational production units for the valorization of biomass by metathesis reaction [11, 12, 17]. Thus, the development of eco-efficient metathesis processes dedicated to the valorization of biomass remains a real economic and technological challenge. In this context, our research group is involved in the development of eco-efficient metathesis processes using robust and highly selective ruthenium catalysts [20, 29], in partnership with companies (IFPEN, Oléon, ITERG, Demeta). The first aspect involves the transformation of terminal olefins from light Fischer–Tropsch cuts derived from forest residues. This process converts these olefins into higher-molecular-weight internal olefins, which serve as precursors for the production of plasticizers or lubricants [30]. The second aspect is oriented toward the transformation of non-refined unsaturated fatty esters from very high oleic sunflower oils (VHOSO) into diesters and unsaturated hydrocarbons, precursors of polyesters, polyamides and high-tech lubricants [31]. Last but not least, continuous flow synthesis has been engaged in the preparation of biosourced odorant molecules and insect pheromones, thus exploiting for the first time homogeneous Z-selective or Z-stereoretentive Ru catalysts [32].
2. Selective metathesis of terminal olefins from biosourced Fischer–Tropsch feeds
To reduce the dependence on oil and limit greenhouse emissions, the French Institute of Petroleum and Renewable Energies (IFPEN) has been involved in an intensive research program on biofuels and chemical intermediates production via biomass transformation. In this context, many efforts have been made to develop a biomass-to-liquid process (BtL) to produce biodiesel and biokerosene from agricultural and forest residues [33] by means of a thermal gasification process [34, 35, 36]. The resulting synthetic gas (syngas) is further converted, after additional purifications, into liquid hydrocarbons using the Fischer–Tropsch process (FTp). The sixty-five percent of heavy hydrocarbons (>C10) are produced and directly used as biofuels (diesel and kerosene) (Figure 4) [37]. The FTp–BtL technology also produces lighter hydrocarbons (e.g., naphtha, including saturated and unsaturated hydrocarbons with five to nine carbon atoms, noted C5–C9), which naturally have a lower market value and whose valorization would impact positively on the overall production costs. In this perspective, olefin metathesis can be successfully employed to convert light hydrocarbons into heavier olefins (C10–C14), which are key intermediates for the production of plasticizers, detergents and lubricants. The coproducts of BtL may thus be used de facto as biosourced useful intermediates (Figure 4).
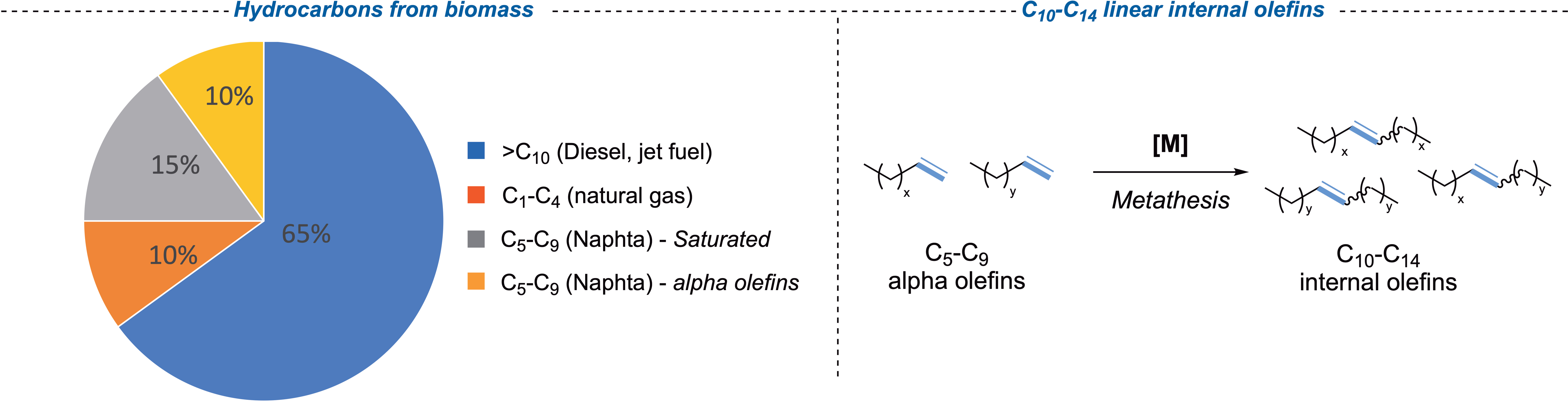
Hydrocarbons from biomass and valorization of lighter unsaturated hydrocarbons by olefin metathesis: an economic challenge.
However, the metathesis reaction applied to terminal olefins, which is often considered as a simple transformation, raises important technological issues when alkylidene-based ruthenium catalysts are employed. These complexes, due to their limited robustness and stability, are highly prone to generate ruthenium hydride species thus promoting isomerization events, even in traces [38, 39]. As a result of this double bond migration, new internal olefins are generated and prone to undergo another metathesis reaction to form undesired side products. In this case, the final outcome is composed of a complex mixture of primary metathesis products (PMP), isomerization (IP) and random metathesis side products (RMP) (Figure 5).
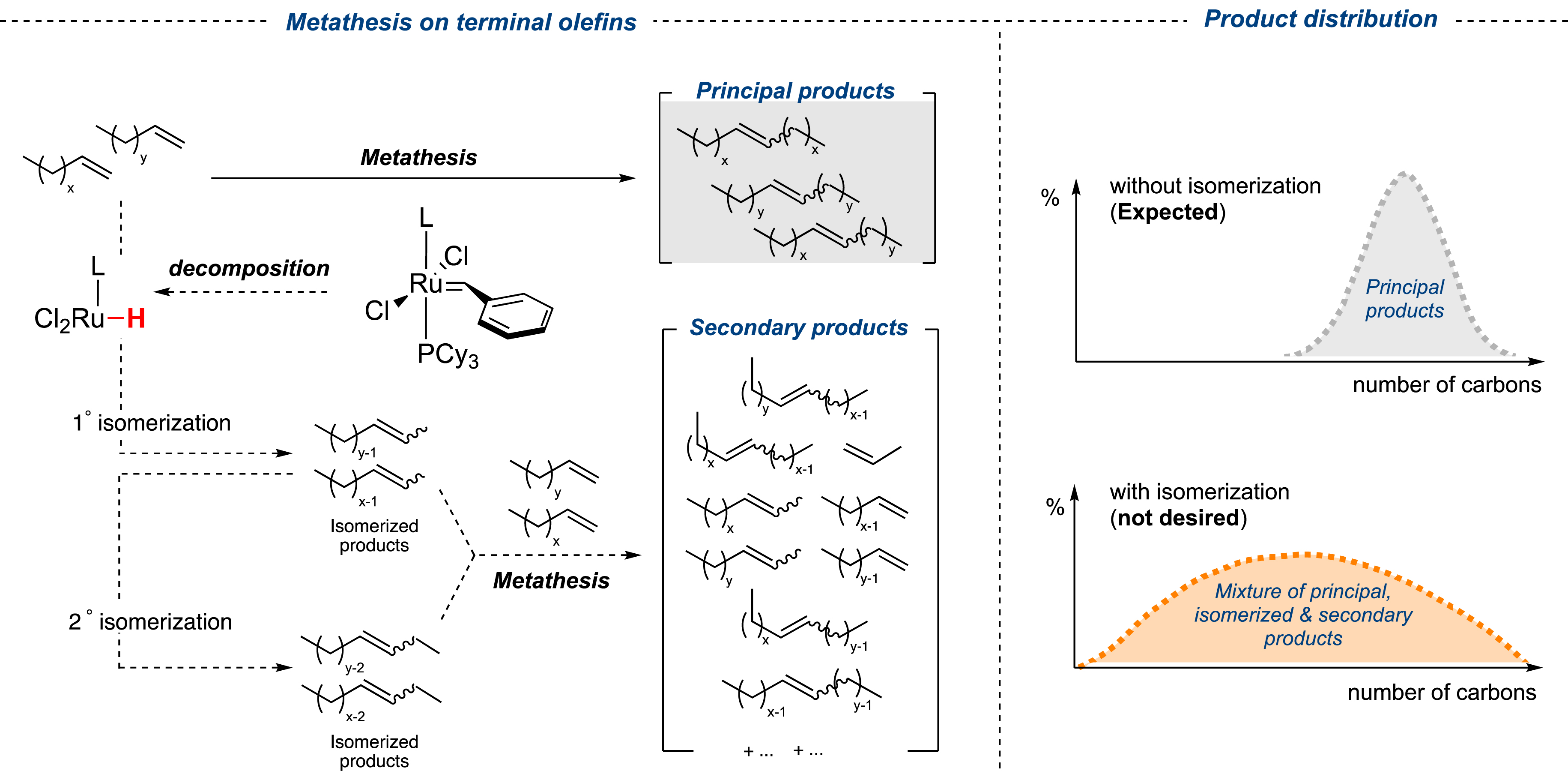
Decomposition of ruthenium catalysts into metal hydrides: the Achilles heel of ruthenium alkylidene for metathesis of terminal olefins.
While the incorporation of additives such as benzoquinone derivatives has demonstrated some effectiveness in trapping ruthenium hydrides within the reaction medium, this approach still presents challenges [39, 40]. For example, considering the toxicity of benzoquinones and their non-negligible costs, this solution may not be always adequate for eco-efficient processes. In parallel, another drawback regarding some of the catalysts concerns their low reactivity, which translates into a consequent/significant increase in catalyst loading [41, 42]. The economic viability of the whole process may then be questioned since, for instance, the costs of the catalysts are excessively high compared to the value of the targeted olefins. Ideally, the transformation of light olefins from the Fischer–Tropsch process should be carried out at ppm-level catalyst loading (50 ppm maximum), thus in the presence of robust metal complexes to avoid the formation of hydride species and use of additional scavengers. With this objective in mind, our research group, in collaboration with Olivier-Bourbigou et al. from IFPEN, made substantial efforts to develop an ecocompatible process to convert lighter olefins (C4–C8) derived from the FTp of forest residues, into heavier internal olefins (C9–C14) [30]. The investigations started with the screening of various catalysts, including commercially available ones (Figure 1), on a benchmark self-metathesis reaction (SM) involving oct-1-ene with a catalyst loading of 0.005 mol% (50 ppm, expressed as mole of Ru per mole of oct-1-ene) in the absence of solvent. Among the studied Ru complexes (more than fifty complexes were developed within the framework of the European FP7 EUMET program), the home-made ruthenium complex Ru-7 [29] demonstrated an interesting reactivity, allowing a conversion of 70% of oct-1-ene into tetradec-7-ene with very high selectivity (98%) and less than 2% isomerization, in stark contrast with the commercially available catalysts Ru-2b and Ru-3b (85% and 80% respectively) (Figure 6) [30]. The robustness of the catalyst was the next parameter to be considered since, after the FTp, the saturated hydrocarbon mixtures contain alcoholic impurities. Remarkably, the selectivity was also maintained under diluted conditions (60 vol% of octane) and was marginally altered in the presence of 10% 1-pentanol (95% vs. 98%) [30].

Self-metathesis of oct-1-ene.
The reason for such a difference in selectivity can be explained by the presence of a modified NHC ligand substituted by a cycloalkane unit by design. This dissymmetric NHC can be prepared in four steps as a tetrafluoroborate salt with an overall yield of 38% (Figure 7A) [29]. In comparison, three synthetic steps were necessary from the inexpensive glyoxal to obtain the symmetrical imidazolinium salt (90% yield), which is the precursor of the 1,3-bis(2,4,6-trimethylphenyl)-4,5-dihydroimidazol-2-ylidene (SIMes) ligand used in many commercial complexes (Figure 1) [43]. Although it can induce very high selectivity, the production of Ru-7 complex on a kilogram scale would be already highly costly. Unsaturated NHCs, such as 1,3-bis(2,4,6-trimethylphenyl)-1,3-dihydro-2H-imidazol-2-ylidene (IMes), are easily accessible in a single step following the well-known Arduengo’s protocol, by simply reacting an aniline with paraformaldehyde and glyoxal in an aqueous acidic medium [44]. Unfortunately, when two aliphatic amines are involved, the expected dissymmetric salt is formed as a statistical mixture of two symmetric salts [45]. Unexpectedly, by mixing an aryl amine (mesitylamine) and an aliphatic amine (cyclododecylamine or cyclopentylamine), an excellent selectivity in favor of the dissymmetric imidazolium salt was observed (>95%), leading to good isolated yields (79–80%) (Figure 7B) [46, 47]. This highly selective multicomponent reaction was extended to the synthesis of various dissymmetric salts (about 30). All of them were isolated in very good yields, ensuring inexpensive access to new precursors of novel dissymmetric unsaturated NHC ligands, including chiral ones [48].
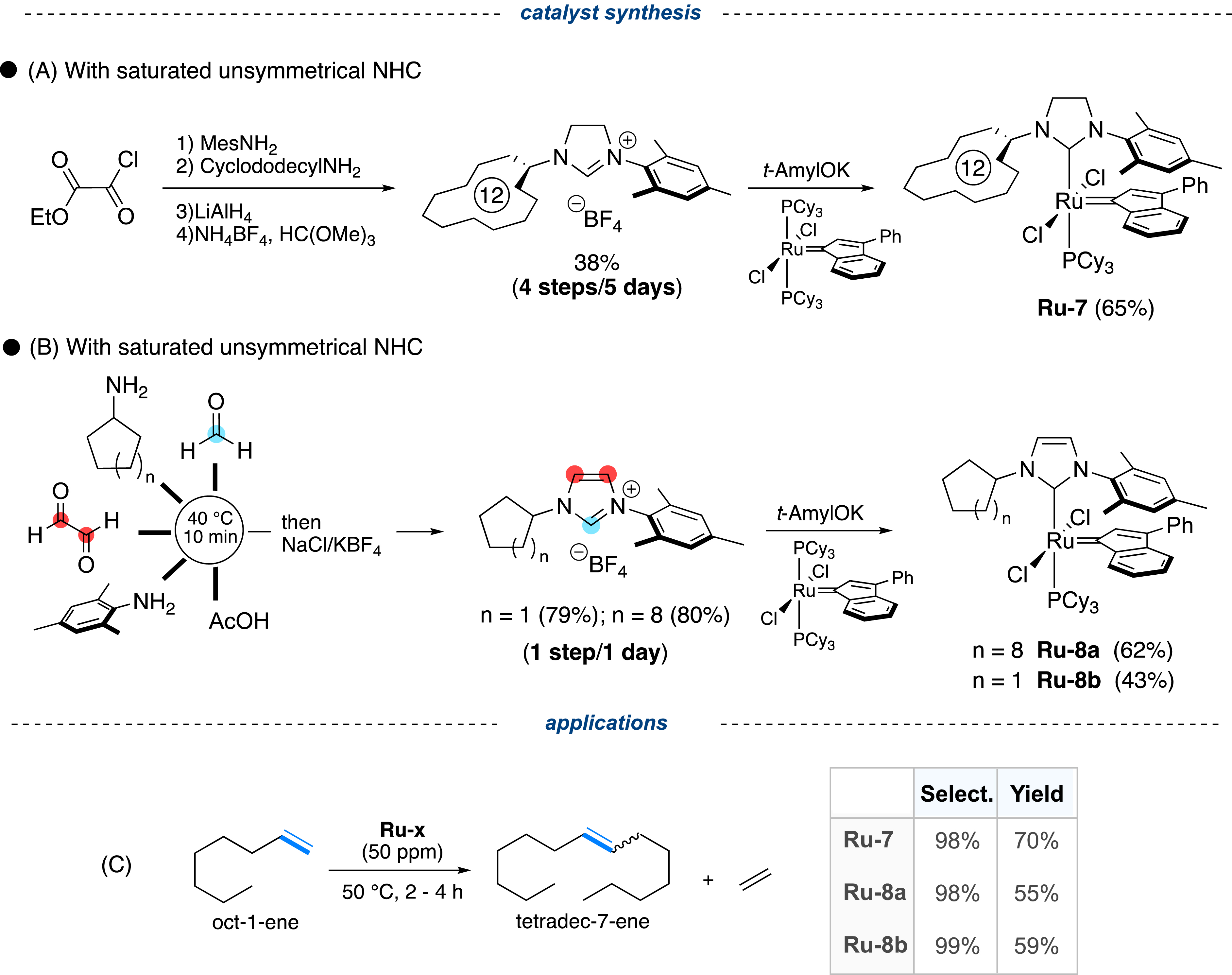
(A) Multistep synthesis of unsymmetrical imidazolinium salts, precursors of saturated N-heterocyclic carbene (NHC) ligands; (B) Multicomponent synthesis of unsymmetrical imidazolium, precursors of low-cost unsaturated NHC ligands; (C) Evaluation in oct-1-ene self-metathesis of the related metathesis complexes.
With a highly competitive route to access dissymmetric unsaturated NHCs in hand, the Ru-8a and Ru-8b complexes, bearing a cyclododecyl and cyclopentyl moiety, respectively, were synthesized and evaluated in the self-metathesis of oct-1-ene (Figure 7C). While the selectivity of the metathesis reaction was retained as expected, a slight alteration of the conversion was also observed (59% vs. 70%). Based on this result, the new Ru-8b complex was selected to convert the light C4 to C8 Fischer–Tropsch cut of 37 wt% olefins, essentially terminal, into mainly C11 and C12 internal olefins. With a catalyst loading of 50 ppm, the new catalyst delivered the expected products with a conversion of 70% and the unprecedented selectivity of 98%, with the distribution of olefins unchanged over time (Figure 8) [30, 49]. The resulting internal olefins (mostly C11, C12) could thus be converted into the corresponding linear alcohols via the OXO process (hydroformylation) and thus potentially used as biosourced raw materials for the production of plasticizers [50].
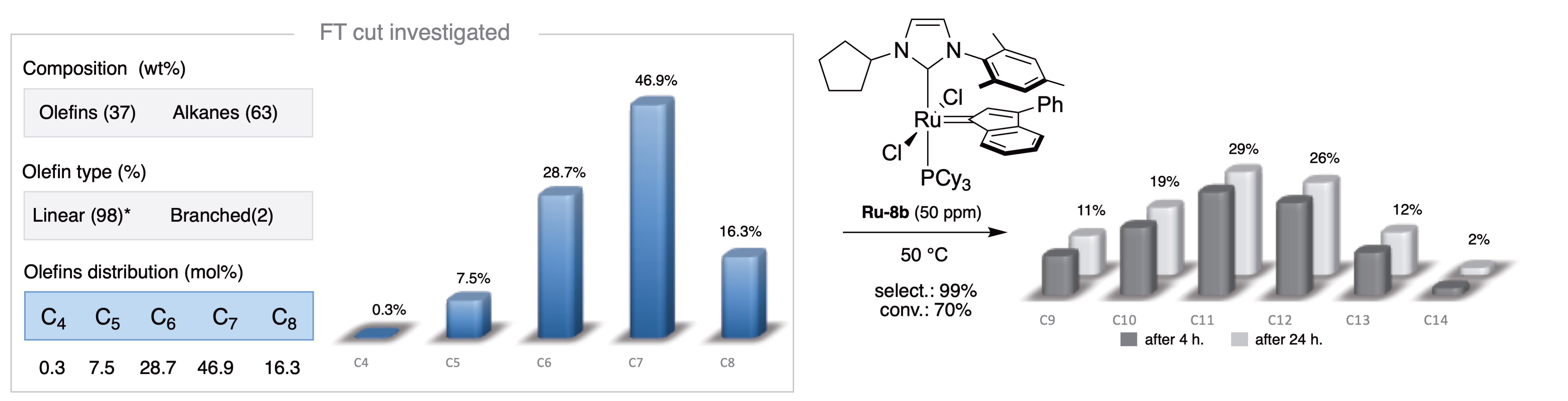
Metathesis on a light hydrocarbon cut (C4 to C8) from the Fischer–Tropsch process on forest residues.
3. Transformation of non-refined unsaturated fatty esters from very high oleic sunflower Oils (VHOSO)
Another biomass transformation of interest involves the olefin metathesis of vegetable oils [17]. Compared to unsaturated hydrocarbons from petrochemicals, vegetable oils contain carboxyl functions which allow a greater modularity in the post-functionalization and therefore to access a multitude of molecular building blocks of interest (alcohols, amides, amines) [18]. The Oleon company has been interested in using olefin metathesis to transform unrefined (Z)-methyl oleate from VHOSO into (E/Z)-2-octadec-9-ene and (E/Z)-3-dimethyl octadec-9-enedioate (Figure 9). These two synthons may be further employed as basic ingredients for the preparation of lubricants, plasticizers, waxes, fragrances, or even monomers for materials (Figure 3) [51, 52].
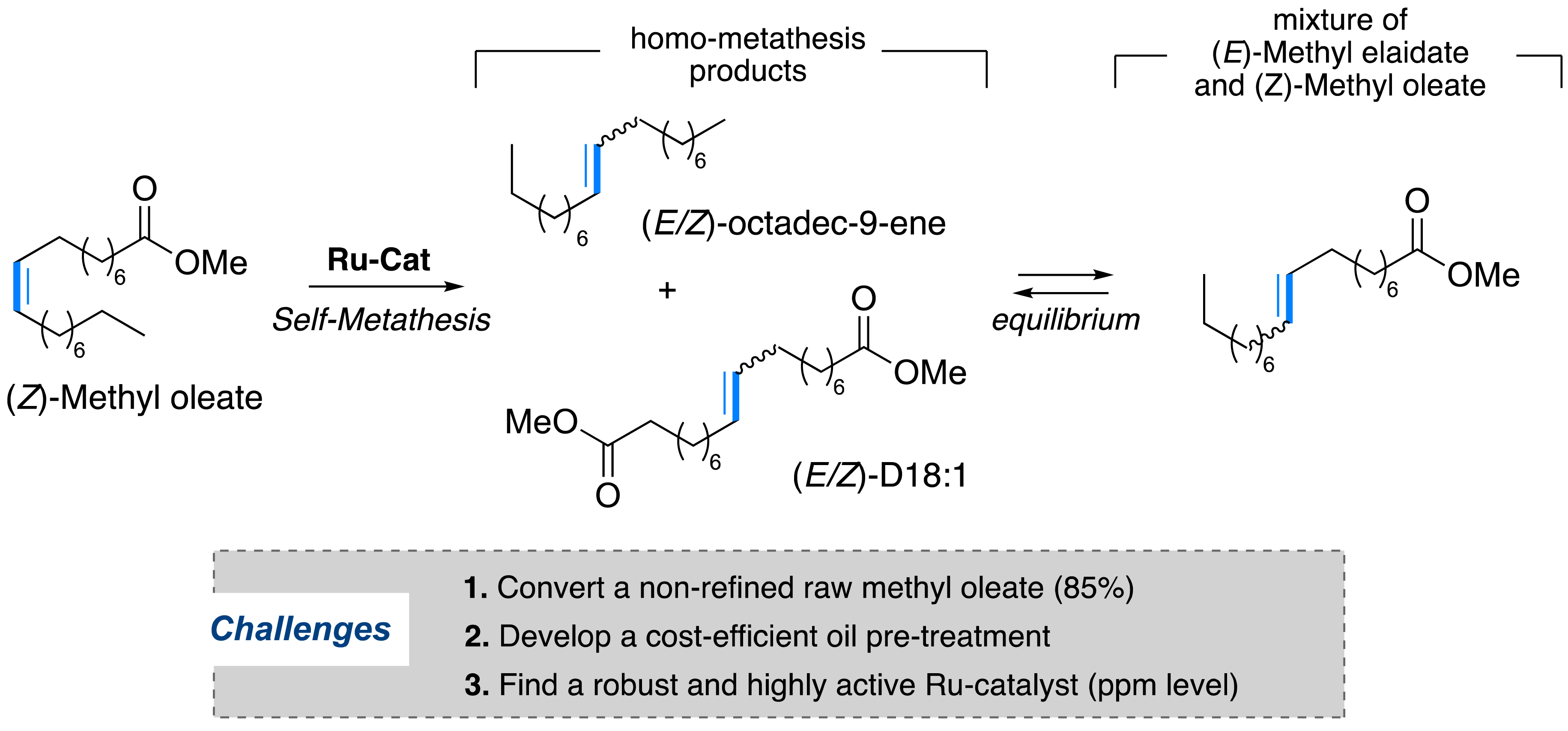
Self-metathesis of non-refined (Z)-methyl oleate for the production of bio-based intermediates.
This self-metathesis reaction involving an internal olefin, albeit simple on paper, requires the removal of certain technological barriers to be attractive, in line with market expectations, with regard to the very low added value of the targeted bio-based intermediates (a few euros/kg). The first barrier concerns the efficiency of the metathesis catalyst; the latter must be active below 0.0005 mol% (5 ppm) and capable of reaching a productive TON close to 100,000. The second barrier is mostly related to the quality of the raw material. The state of the art relates to a few examples where second-generation metathesis catalysts such as Ru-1, Ru-2b, or Ru-4 allowed the reaction equilibrium with only 1 ppm of catalyst loading, corresponding to TONs ranging between 250,000 and 440,000 [53]. However, this remarkable efficiency was achieved on the transformation of highly pure methyl oleate (>99%), thus requiring distillation and/or prefiltration on alumina. Raw materials of such quality are unsuitable for industrial scale since the purification processes on a larger scale would be highly costly. Our group has succeeded in refining the oil to a purity of 97% on a kilogram scale (in collaboration with ITERG). Albeit efficient, this process involves a double cold crystallization, which is not feasible on a larger scale. The metathesis reaction may be therefore economically viable only if it is performed on unrefined methyl oleate with a purity of only about 85% [54]. The catalyst should be robust, efficient, and productive in the presence of impurities (Figure 11). The key solution lies in the use of a very robust catalyst but above all, in the implementation of an eco-efficient pre-treatment process of the unrefined raw material, which would allow the effective elimination of impurities that are deleterious to the catalyst. Over the last decade, a few pre-treatment processes have been developed by different companies operating in the field (e.g., Elevance, Croda, Arkema). However, they suffered either from a lack of efficiency or an excessive cost of the catalyst, leading overall to a non-negligible loss in raw material during the filtration processes [17, 55]. Our group, in collaboration with industrial partners (Oleon, ITERG, and Demeta), worked on the development of an economically viable catalytic process for the transformation of unrefined methyl oleate at 85% purity [31].
To determine the adequate pre-treatment, it was crucial to identify the compounds present in the unrefined raw material (here sunflower oil) likely to poison the catalyst. As depicted in Figure 10, it was possible to classify those impurities into three main groups: (1) those naturally present in the vegetable oil (sunflower lecithin, tocopherol acetate and sterols); (2) the compounds resulting from the hydrolysis and transesterification of the oil (oleic acid, glycerides, glycerol, methanol, and water), and (3) the residues resulting from the oxidation of the oil (stand oils, n-nonanal and peroxidized methyl ester). As shown in Figure 11, only glycerides, oleic acid, nonanal, and peroxidized methyl ester (added up to 10% in mass) had a detrimental effect on the self-metathesis of refined methyl oleate catalyzed by the M71 Ru-6a complex (500 ppm) [56], where reaction equilibrium was reached in only 5 min in the absence of any additive, while normally this transformation is highly slow or never complete.
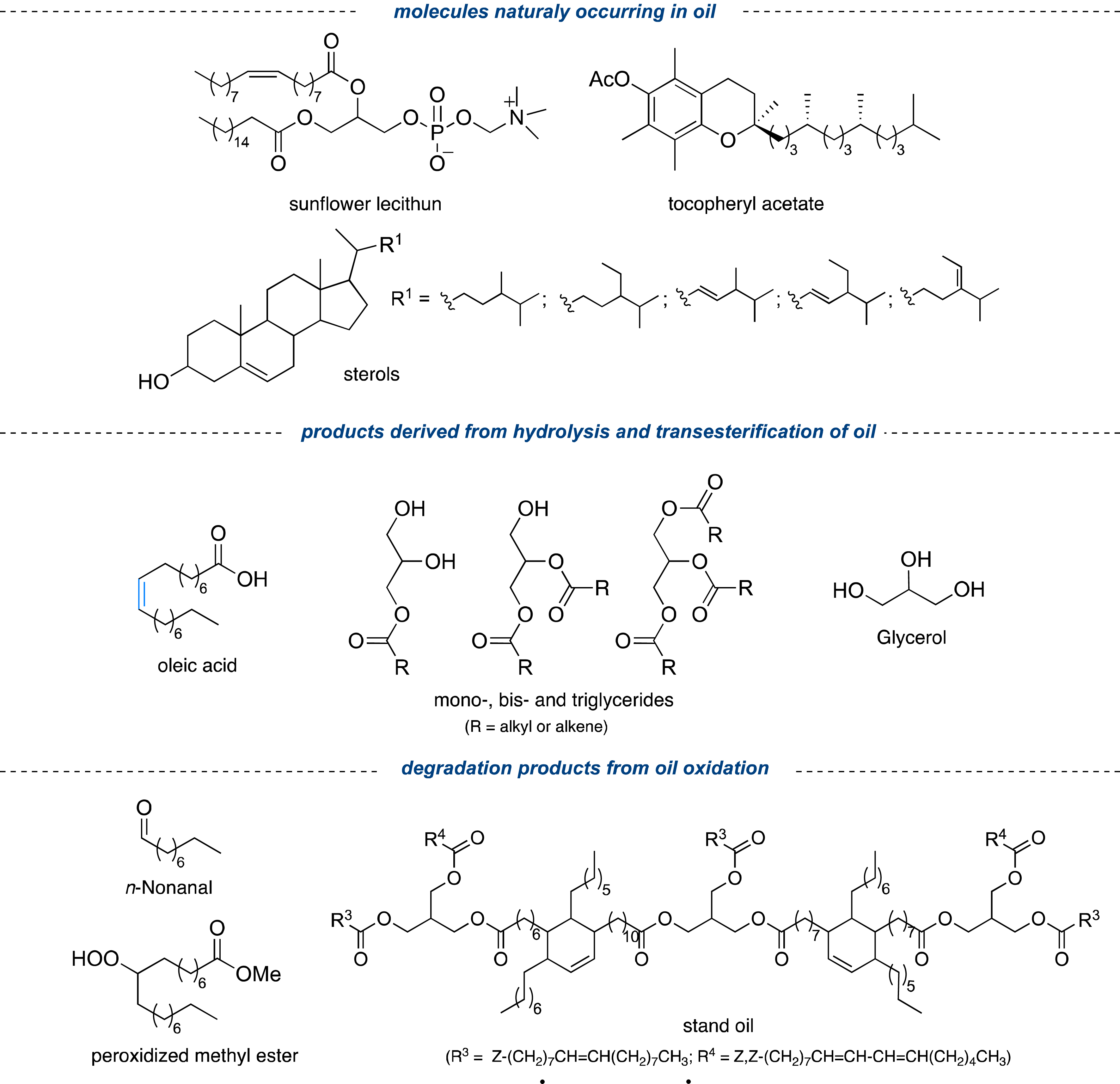
Compounds frequently detected in non-refined unsaturated fatty esters that can poison the metathesis catalyst.
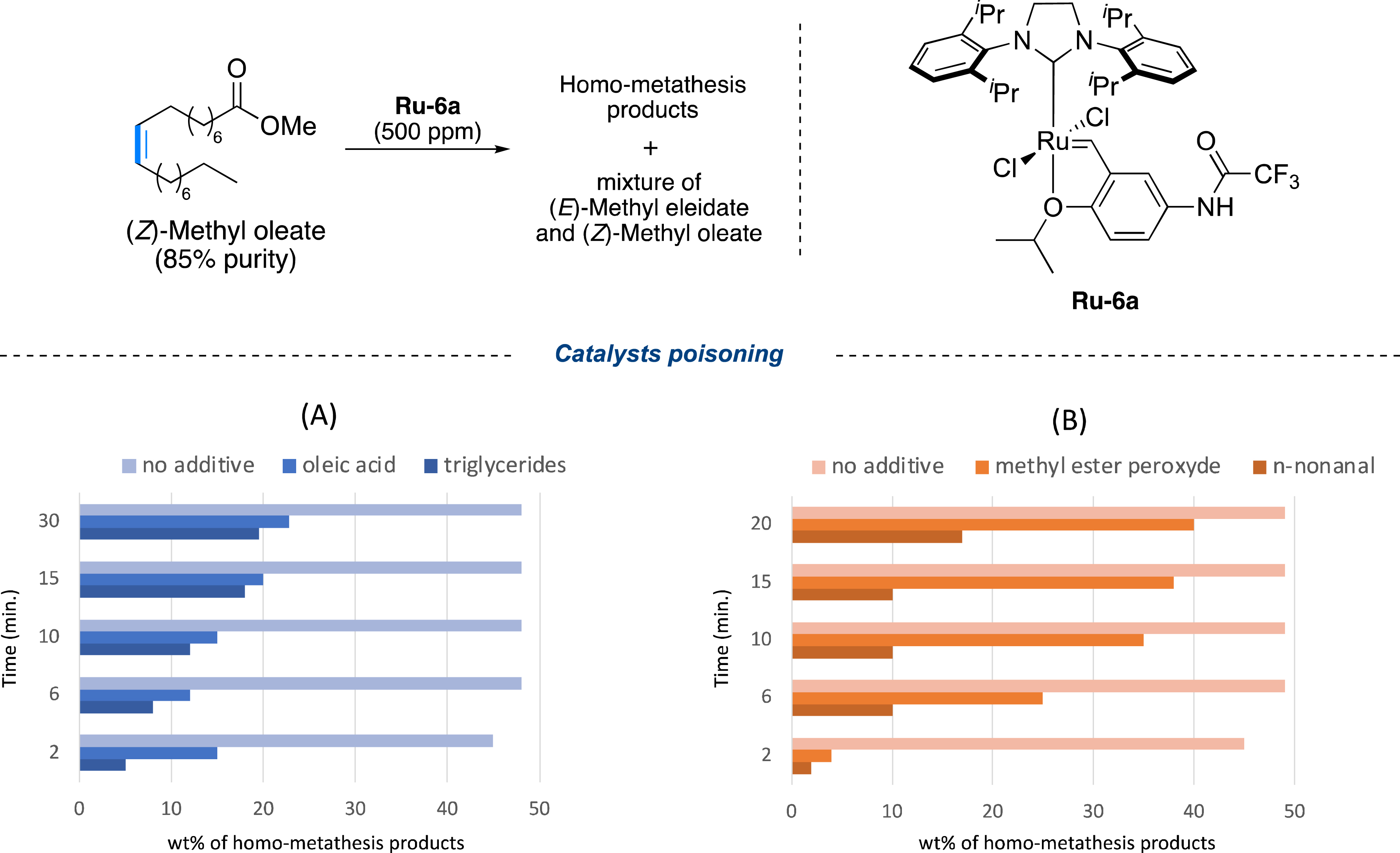
Effect of catalyst-killer molecules in the self-metathesis of (Z)-methyl oleate: (A) from oil hydrolysis and (B) from oil oxidation.
Our group turned its attention to unrefined methyl oleate at 85% purity to identify the ideal pre-treatment that would inhibit the deleterious effect of these impurities on catalyst efficiency. Our choice was quickly made for bleaching earths, which are frequently used in the refining of vegetable oils to eliminate pigments (chlorophyll, carotenes) and other impurities (e.g., traces of metals or oxidation products) [57]. As soon as the first test was carried out, beneficial effects were noticed when 10 wt% of tonsil® 110FF were added (Clariant, composition: SiO2 75.5%, Al2O3 12%, Fe2O3 2.4%, CaO 0.4%, MgO 1.4%, Na2O 0.3%, K2O 1%), since the equilibrium was reached with a catalyst loading of 0.02 mol% (200 ppm), whereas three times more catalyst was required in the absence of treatment (Figure 12). If the use of tonsil® 110FF is combined with a thermal pre-treatment (a well-known process to drastically reduce the naturally present peroxides in fatty esters), equilibrium is reached after 1 h in the presence of only 0.0005 mol% (5 ppm) of Ru-6a complex only. However, the abrasive character of the tonsil®, which is essentially composed of silica, represents an important deterioration risk of the stainless steel reactors. With a significant increase in contact time between tonsil® 110FF and unrefined methyl oleate (from 15 to 120 min) prior to the addition of the catalyst, it was possible to reduce the amount of tonsil® 110FF by 1 wt% without any loss of efficiency. Under these optimal conditions, the limits of the catalytic process were reached with a catalytic loading of 0.0001 mol% (1 ppm), representing an unprecedented maximum TON of 744,000 and a productive TON approaching 360,000, despite the use of unrefined feedstock.
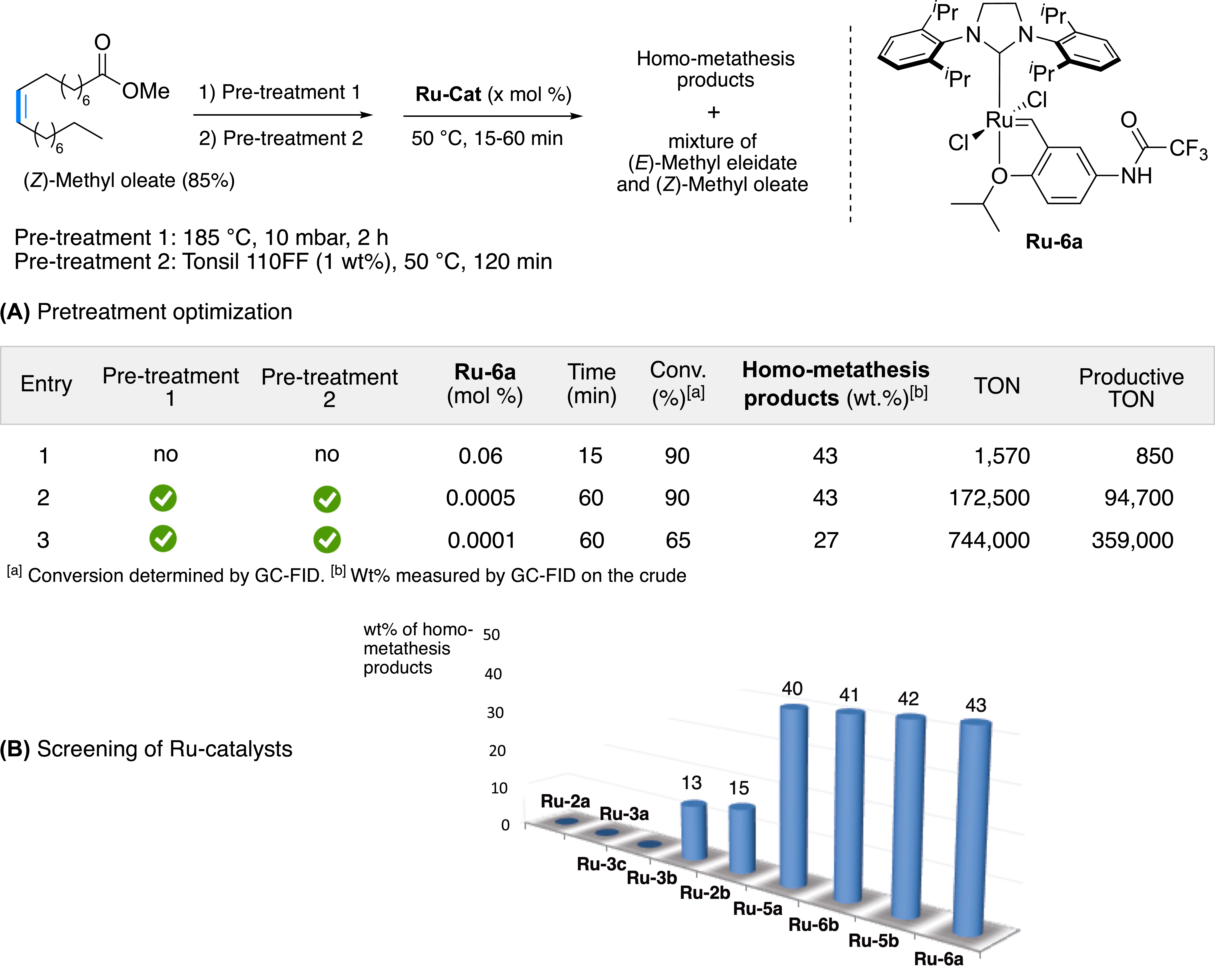
(A) Pre-treatment optimization and subsequent self-metathesis of (Z)-methyl oleate. (B) Screening of commercially available Ru catalysts.
After the pre-treatment optimization of raw materials, a screening of various commercially available catalysts was carried out showing significant differences in their reactivity profiles (Figure 12B). The first- and second-generation phosphine-based ruthenium complexes Ru-2a, Ru-2b, Ru-3a, Ru-3b and Ru-3c proved to be inefficient, most certainly due to too fast a degradation in the reaction medium. Hoveyda-type catalysts showed higher robustness than the phosphine-based ruthenium complexes, particularly complexes with a sterically hindered NHC SIPr ligand (Ru-5b and Ru-6a). The Ru-6a catalyst was selected for the scale-up of the catalytic process, performed at ITERG and then by the Oleon group (Figure 13). The first test was performed on 1 kg of raw material using 0.01 mol% of Ru-6a complex. Under these conditions, the reaction equilibrium was reached after 15 min. A test on 3 kg and a catalytic load of 0.0005 mol% provided a conversion of 88% of methyl oleate after 1 h, consistently with the experiments performed on a laboratory scale. Based on these results, two pilot tests were performed on a 300 L reactor (Figure 13). Two batches of 200 kg of unrefined feedstock were successfully transformed, with a conversion rate of 88% at equilibrium (TON of 174,000) and excellent productivity (productive TON of 95,000), thus demonstrating the simplicity and robustness of the pre-treatment and overall catalytic process [31].
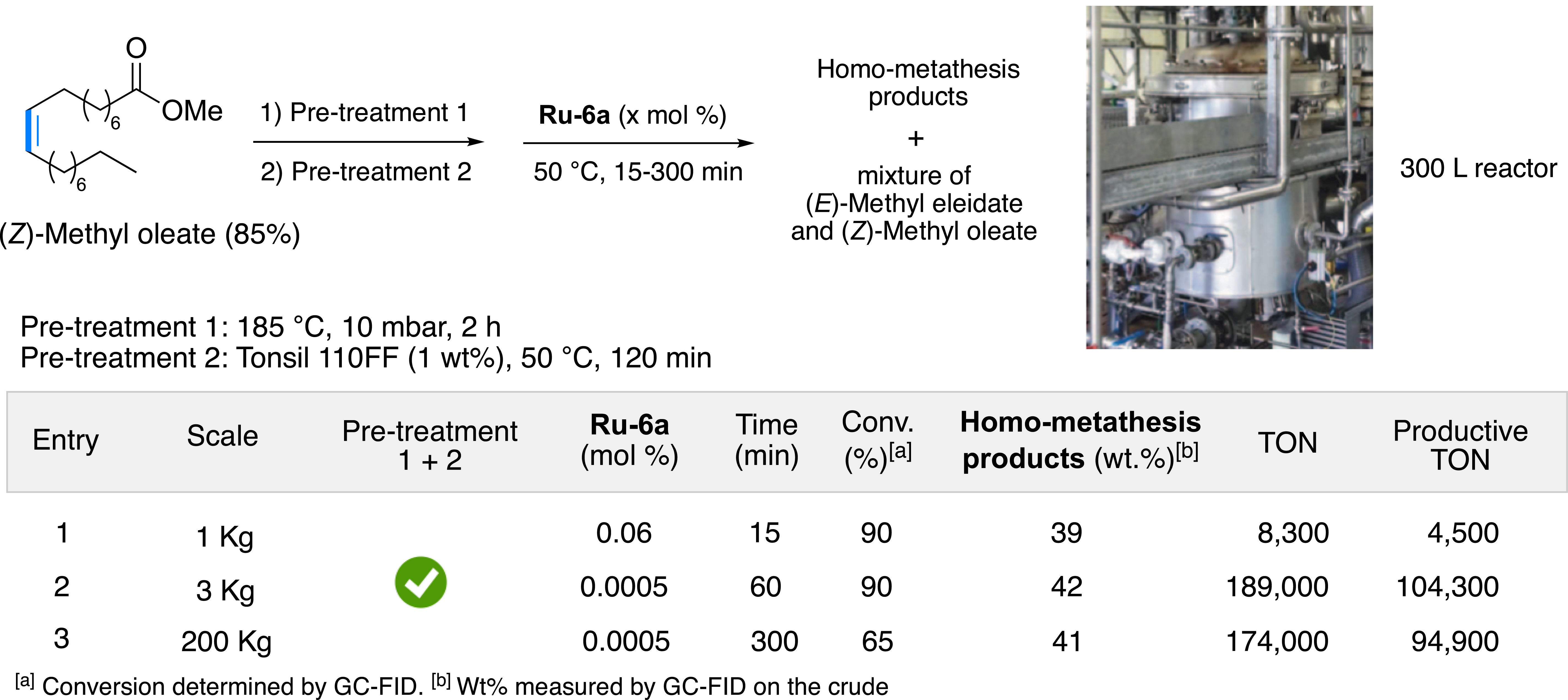
Scaling up of the catalytic process.
In order to avoid the drawbacks related to the high cost and reduced performance of metal catalysts toward raw biosourced materials, our group then focused in 2019 on the development of a straightforward protocol for the in situ generation of an active ruthenium complex, combining inexpensive commercially available reagents such as (p-cymene)ruthenium(II) chloride dimer ([Ru(p-cymene)Cl2]2), SIPr·HCl, and n-BuLi in toluene [58, 59]. The resulting 18-electron species Ru-9 is formed and directly used without any purification step for a metathesis reaction in the presence of activators such as alkynes, cyclopropenes, and diazoesters. The diazoester A1 was identified to be the most efficient activator to convert the (Z)-methyl oleate (99% purity) into highly valuable mono-unsaturated diester and hydrocarbon with a catalyst loading under 0.01 mol% (100 ppm) (Figure 14, entry 1). This expedient protocol was applied to 2 kg-scale self-metathesis of a non-refined technical grade (Z)-methyl oleate (85% purity) using only 0.04 mol% (400 ppm) of catalyst loading (Figure 14, entry 2) [58].
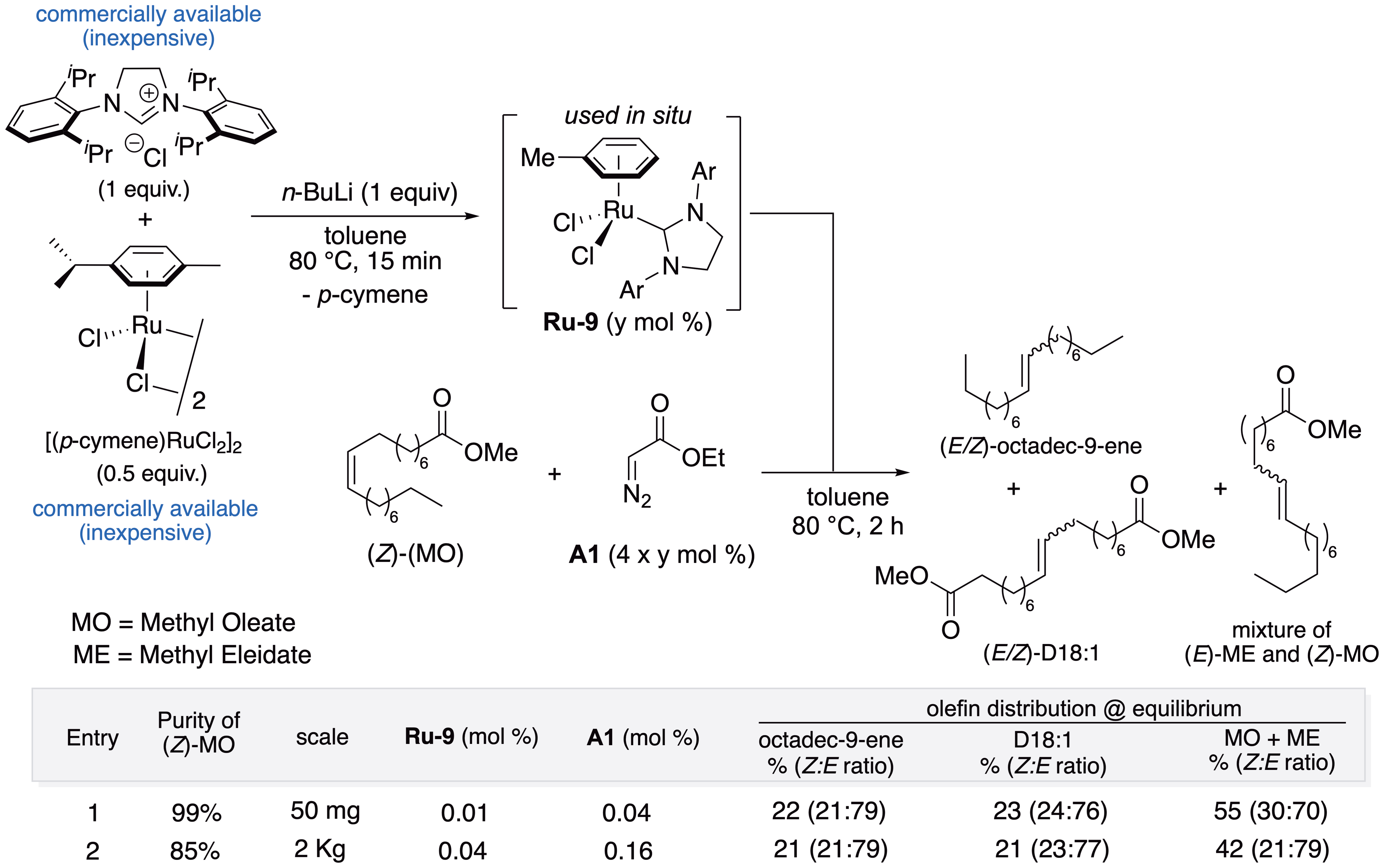
In situ generation of Ru-based metathesis catalyst. Application to self-metathesis of (Z)-methyl oleate (up to 2 kg).
4. Continuous flow Z-selective olefin metathesis
As mentioned previously, vegetable oils have the advantage to be easily post-functionalizable thanks to the intrinsic carboxyl function. This feature provides access to a wide range of building blocks of interest such as amides, amines, alcohols and ketones [18]. It is worth mentioning that alcohols and ketones can be used as starting materials to synthesize highly desirable insect pheromones [60] or macrocyclic odorant molecules [61] via olefin metathesis. However, many of these valuable molecules have an alkene group with a strict Z geometry, giving them a three-dimensional architecture that matches with their biological activity. Since 2013, important breakthroughs have been achieved in controlling Z-selectivity during the cross-metathesis and the macroring closing metathesis, thanks to the development of efficient Z-selective and Z-retentive ruthenium catalysts based first on NHCs [62, 63, 64, 65] and more recently on CAACs (Figure 15) [66]. Unfortunately, besides their exceptional utility, these catalysts remain extremely sensitive to oxygen, air, and moisture, therefore they require the use of a glovebox to perform the metathesis reaction, which could potentially limit their applications in industrial settings.
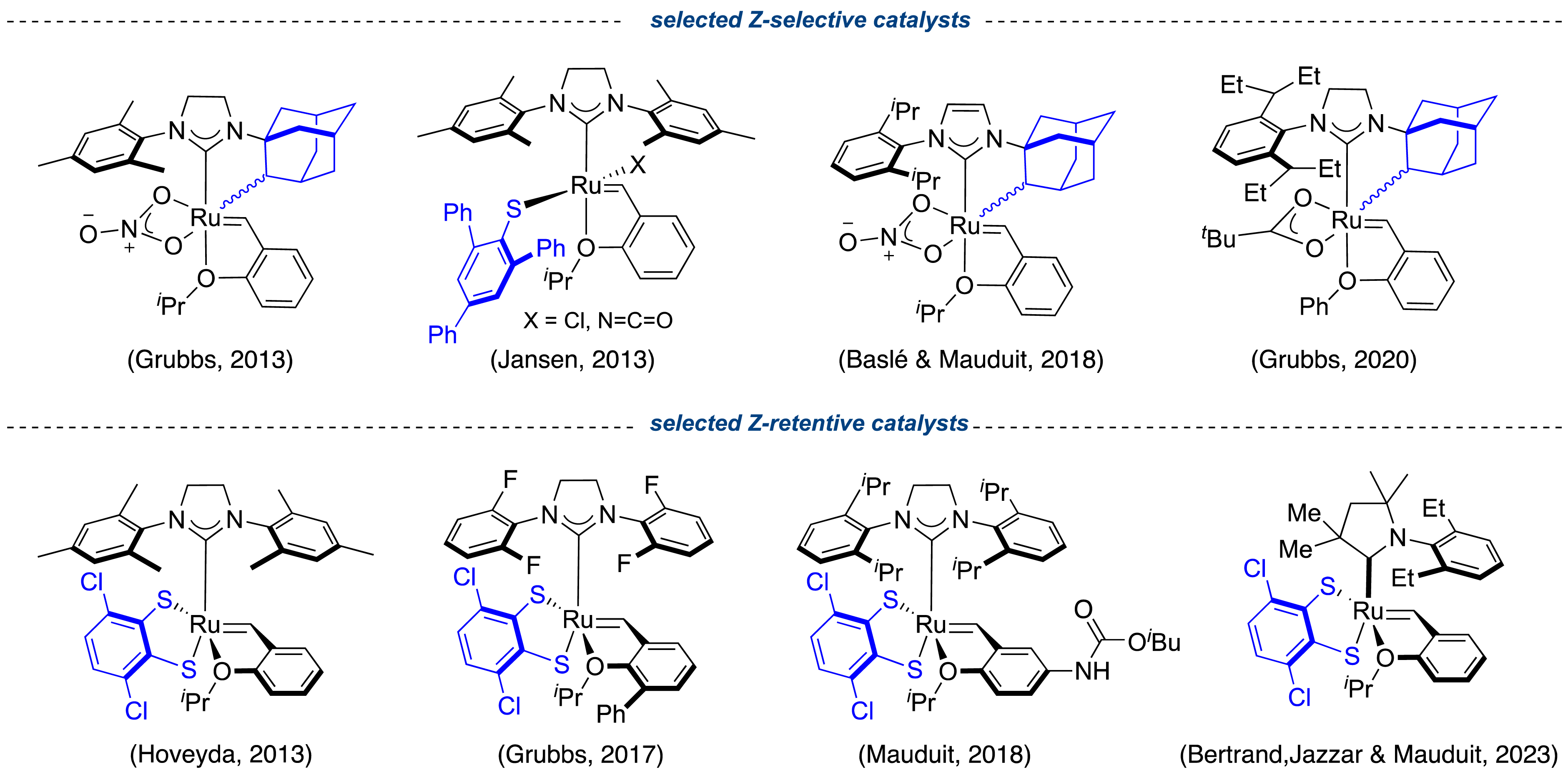
Top: Selected examples of Z-selective catalysts; bottom: Z-retentive catalysts.
To overcome the problems related to the manipulation of highly sensitive catalysts, our team first developed a highly practical in-situ protocol which is able to promote the Z-selective metathesis without the need of a glovebox (Figure 16) [67]. By reacting commercially available catechodithiol L1 with diethylzinc, then adding the Hoveyda catalyst Ru-5a, a solution of the expected dithiolate catalyst Ru-10 was obtained. This catalyst was then added to an alkene in the presence of but-2-en-1,4-diol to give the desired Z-product in high yield and excellent stereochemical purity. This operationally simple protocol was successfully applied to the synthesis of valuable functionalized molecules (Figure 17).
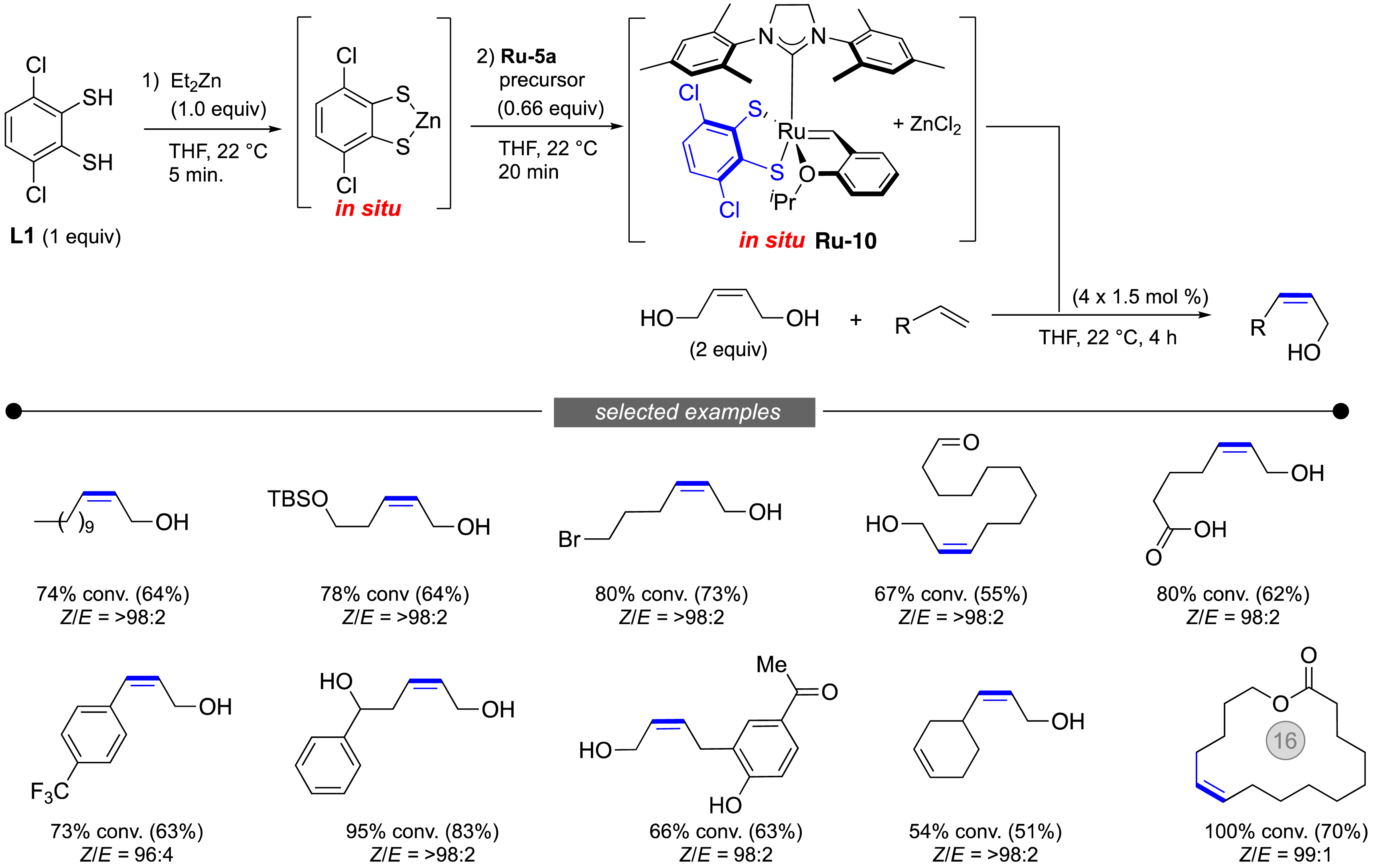
In situ generation of Z-retentive catalysts and stereoretentive olefin metathesis.
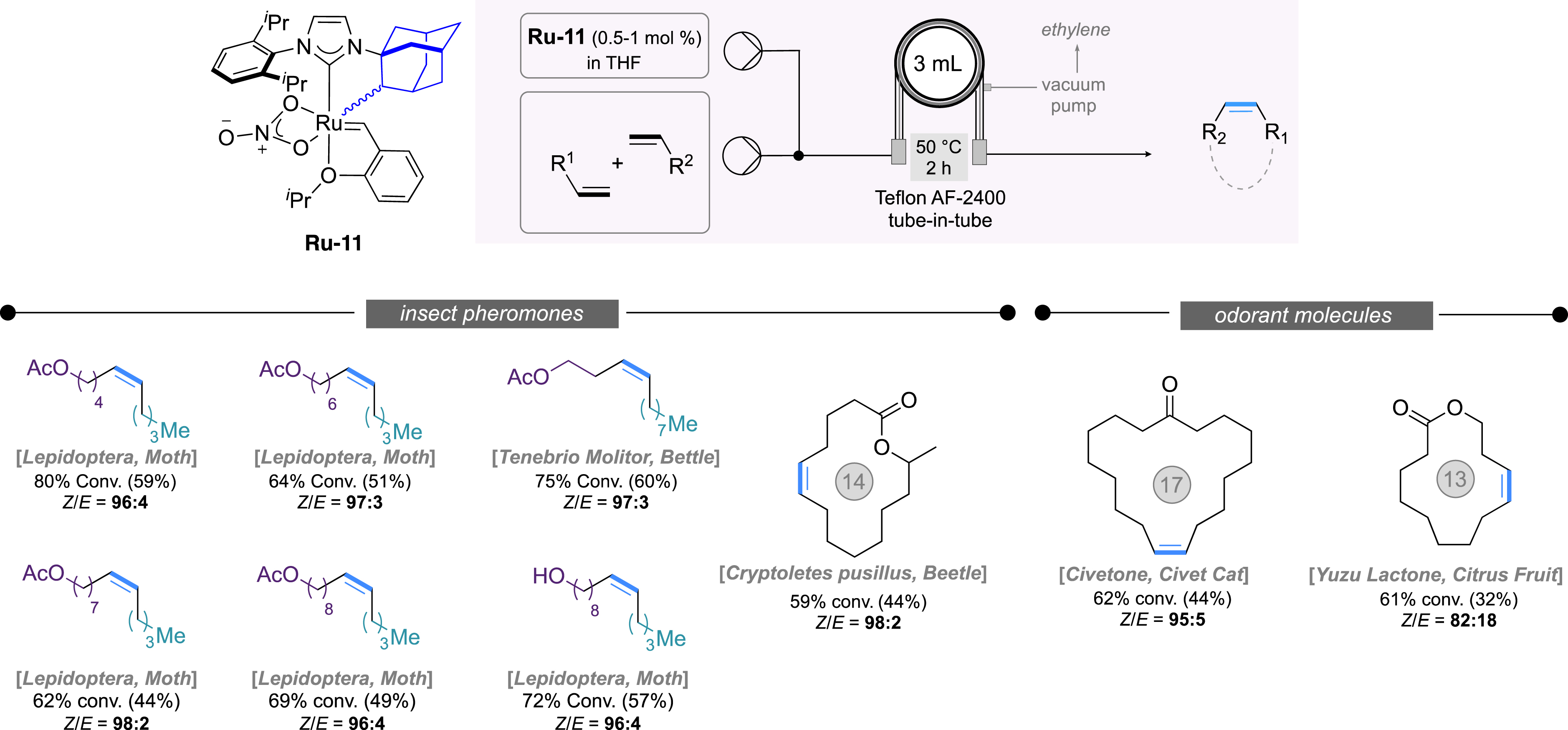
First developments in continuous flow Z-selective metathesis: selected applications in the synthesis of (biosourced) insect pheromones and odorant molecules.
In a second stage, we focused on the implementation of the first Z-selective metathesis in continuous flow process in collaboration with the Browne research group (UCL School of Pharmacy, London) [32, 68]. By using a Teflon AF-2400 tube-in-tube semi-permeable membrane reactor, various semiochemicals (i.e, pheromones), acting as potential biopesticides against pest insects, were efficiently synthesized with high Z-selectivity, even at 6 mmol scale, thanks to the robust cyclometallated complex Ru-11 featuring a bulky unsaturated NHC. This method was also successfully applied to the production of civetone and yuzu lactone, two highly desirable macrocyclic odorant molecules. Interestingly, the in situ generation of stereoretentive cathechodithiolate catalyst Ru-10 (vide supra) [69] also proved to be highly efficient in continuous flow affording the biosourced Tenebrio Molitor pheromone in 49% isolated yield and with a Z/E ratio above 99:1 (not showed here).
On the other hand, the search for more stable, easily accessible and highly efficient ruthenium catalysts led to the design of new ligands along with the investigation of other possible modifications on the hemilabile styrenyl ether ligand [66]. Our group, in collaboration with G. Bertrand and R. Jazzar (UCSD), very recently developed a new ruthenium-based catalyst featuring a CAAC ligand and a “Blechert-type” styrenyl ether [70]. The combination of those two elements led to the synthesis of an organometallic species which summarizes the stability offered by the CAAC and the high initiation rates peculiar for this styrenyl species. As a result, the Blechert-type catalyst Ru-12 was able to perform a series of metathesis reactions at low catalyst loading (down to 0.005 mol%) [66]. Particularly, the synthesis of civetone was successfully achieved in 81% isolated yield and with high purity (99%) (Figure 18). Further developments will concentrate on the synthesis of the corresponding catechodithiolate Ru complex for promoting Z-metathesis at very low catalyst loading.
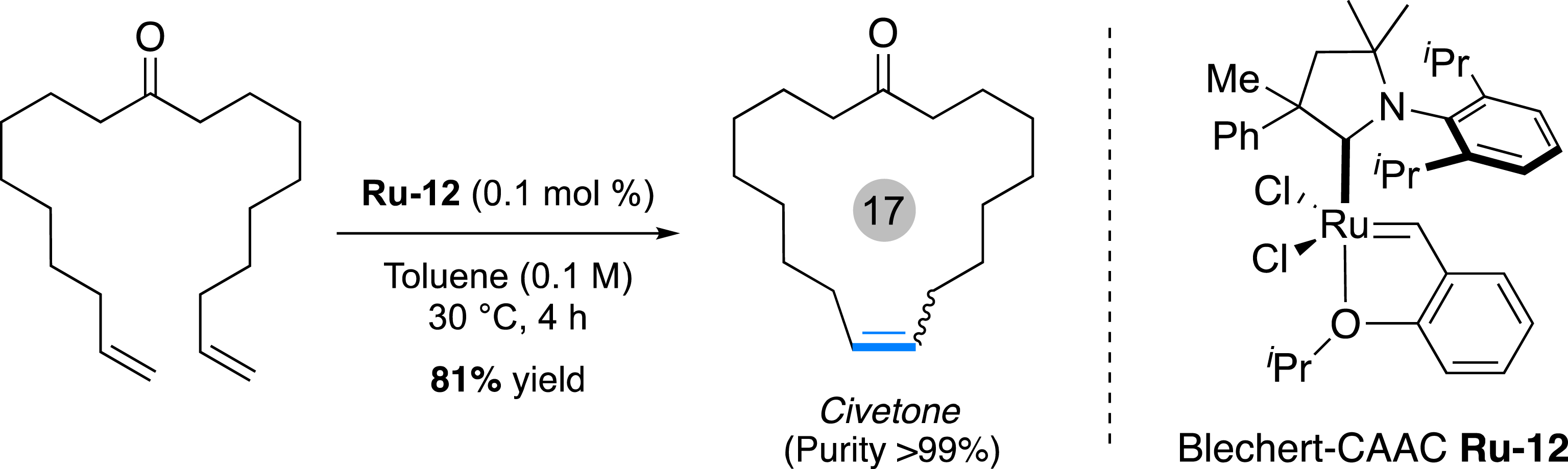
Macro-ring closing metathesis catalyzed by Blechert-type CAAC–Ru complex.
5. Conclusion
Olefin metathesis rightly consolidated its position as an irreplaceable versatile synthetic tool for chemists offering, when applicable, numerous advantages such as reduced reaction time, simplification of synthetic routes, and a lower carbon footprint. Today it seems relatively straightforward to envisage applications of olefin metathesis in biomass valorization or to convert renewable raw materials into molecules of interest. However, due to the very low added value of the targeted products, the implementation of metathesis reaction on an industrial scale requires overcoming additional barriers to become highly competitive. To meet this challenge, it is necessary to design not only more robust, more selective and, above all, less costly catalysts, but also to develop ingenious processes to drastically reduce catalyst loading. Addressing these challenges, we have explored the transformation of biosourced olefins. In the case of unsaturated hydrocarbons coming from forest residues, use of innovative ruthenium catalysts based on easily accessible dissymmetric unsaturated NHC ligands, has enabled the efficient conversion of light linear terminal olefins into linear heavier internal olefins. These olefins serve as valuable precursors for plasticizers. The novelty lies not only in the reduced costs of the catalyst and its use at very low loadings (0.005 mol%), but also costly and toxic additives were not necessary to limit the formation of by-products. Similarly, in the case of unsaturated fatty esters from vegetable oils, the development of a pre-treatment based on bleaching earth, coupled with the use of a robust catalyst, allowed an efficient conversion of unrefined methyl oleate (85% purity) into diester and unsaturated hydrocarbons, precursors of polymers and lubricants. Unprecedented productivities have been achieved (productive TON up to 360,000), despite the presence of impurities that could poison the catalyst. The successful performance on 200 kg pilot scale paved the way for potential applications at industrial scale, thus impacting the production of fragrances, pheromones and biofuels. Last but not least, by combining Z-stereoselective Ru catalysts with continuous flow processes, the production of molecules of interest such as odorant molecules for perfume industry or semiochemicals against insect pests can be achieved efficiently. While increasing process productivity and reducing waste, the continuous flow metathesis reaction can make a significant overall impact on production costs.
Declaration of interests
The authors do not work for, advise, own shares in, or receive funds from any organization that could benefit from this article, and have declared no affiliations other than their research organizations.