1 Introduction
Oxidation reactions are of great importance for the production of bulk chemicals, specialties and fine chemicals. In addition, ‘destructive’ oxidative bleach processes play a major role for the pulp and paper production, textile pre-treatment, waste water treatment and industrial and domestic laundry processes. These bleach processes are directed to destroy chromophores, thereby increasing the brightness of the respective substrate. Although chlorine based bleach processes play still a significant role, hydrogen peroxide and oxygen are increasingly favored as environmentally acceptable and non-toxic bleaching agents. Bleach processes consume already today the vast majority of the hydrogen peroxide produced worldwide [1]. Bleach is of special interest in alkaline media, in which industrial and domestic bleach is typically carried out. Although hydrogen peroxide and oxygen are potent oxidants in a thermodynamic sense, their action is kinetically hindered. The search for catalysts being able to effectively catalyze hydrogen peroxide or even molecular oxygen based bleach reactions has been a major focus for almost two decades (for recent reviews see: Refs. [2,3]).
In laundry, bleach is mainly directed to destroy unwanted stains on the fabric, in order to achieve the intended cleaning effect. In a second type of application, bleach might be applied to destroy dyestuff in solution, in order to prevent discoloration of acceptor fabrics by migrating dyestuff bleeding from colored textiles (dye transfer inhibition – DTI). Laundry detergents typically contain percarbonate or perborate salts as bleach systems. These peroxy salts release hydrogen peroxide, when being dissolved in the wash liquor. The bleach activity of hydrogen peroxide is sufficient at temperatures above 60 °C. Activator systems, for example based on N,N,N′,N′-tetraacetylethylene diamine (TAED), have been developed and are applied in many laundry detergents (for review see: Refs. [4,5]). Activators release a peroxy acid after perhydrolysis (peracetic acid in case of TAED), which typically shows reasonable bleach results at 40 °C and above. However, the washing temperatures in many parts of the world are significantly below 40 °C and there exists a widespread trend in Europe to safe energy by using lower washing temperatures. This clearly drives the research for catalytic systems being able to effectively activate hydrogen peroxide or even molecular oxygen at low temperatures.
Catalysts based on iron and manganese complexes are in the center of research. Both transition metals are acceptable from an environmental and toxicological point of view. The fact that many manganese and iron based enzymes exist in biology reinforces the optimism of finding effective catalysts for bleach reactions.
However, the use of such catalysts for the laundry application is often hampered by the specific process conditions. The catalysts have to be active at low temperature, in an aqueous medium at alkaline pH. Many catalysts, which show a reasonable performance in organic solvents, fail under these conditions because of a relatively low thermodynamic complex stability resulting in a fast decomposition due to the formation of manganese dioxide or iron hydroxides under alkaline conditions. Moreover, the limited oxidative robustness of many ligands forbids their use as bleach catalyst. To our experience, out of 100 different ligand systems arbitrarily selected from the academic literature, manganese or iron complexes of about 98 would not give a noticeable activity in the laundry process, either because of the low intrinsic activity or the insufficient stability.
The chromophores of bleachable target stains are often based on polyphenols or carotenoids. Typical polyphenolic stains include tea, coffee, and red wine. Carotenoids can for example be found in tomato and carrots. A high activity on these types of stain is a major requirement for any bleach catalyst. The most prominent example of a catalyst meeting the required stability and activity criteria is a dinuclear manganese complex containing 1,4,7-trimethyl-1,4,7-triazacyclononane as a ligand (Mn–Me3TACN, Fig. 1) [6]. This catalyst is not only very active in stain bleaching in laundry but also shows interesting activities in epoxidations [6–9], dihydroxylations of alkenes [10], alcohol oxidation [11], C–H activation [12], and oxidation of phenols [13]. Mn–Me3TACN was the first catalyst employed in commercial detergents. However, the high stain-bleaching activity was accompanied by an enhanced damage of cellulose fibers and increased fading of certain textile dyes, and the laundry products hence had to be withdrawn from the market.
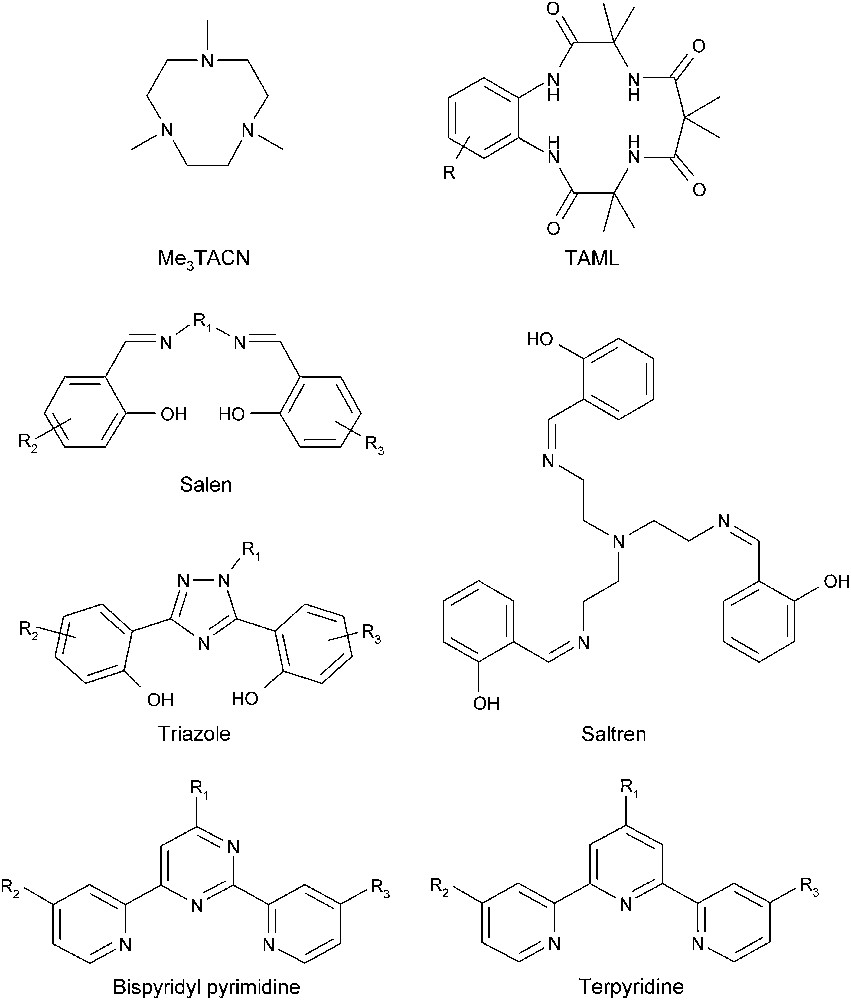
Typical ligands of bleach catalysts.
Another class of catalysts, especially designed to meet the stability criteria, are Fe complexes with macrocyclic tetraamido ligands (Fe–TAML, Fig. 1) [14]. These catalysts are extremely efficient in oxidizing structurally quite different dyes in solution and many papers have been published on the potential use in pulp and paper bleaching as well as waste water treatment [15–18]. In laundry, these catalysts are claimed as potential dye transfer inhibition (DTI) catalysts preventing the staining of white or lightly colored textiles by decomposing migrating dyestuff [19].
Over the last years, our group has studied quite different classes of transition metal complexes in order to assess their potential use as catalysts for stain bleaching or dye transfer inhibition in laundry. These include Mn and Fe complexes of salens and other imine-type ligands [20–23], bis-(o-hydroxyphenyl)-1,2,4-triazole ligands [24], bispyridyl pyrimidines [25] and terpyridines [26–28] (Fig. 1). In this paper we review important aspects of our research. We illustrate, how highly effective dye transfer inhibition catalysts can be designed and optimized to meet the requirements of the laundry process. Terpyridine–manganese complexes represent a class of very potent stain-bleaching catalysts [27]. Based on model systems we show herein, how the parameters influencing activity can be understood and how the activity of these catalysts is optimized. For a broad market penetration any catalyst has to show, in addition to a proper bleach performance, a high level of dye and fiber safety under a variety of very different application conditions. We briefly summarize basic aspects of undesired side effects of catalytic bleach systems.
2 Catalysts for dye transfer inhibition
2.1 Requirements for DTI catalysts
Textile dyes often bleed out of garments during washing and these fugitive dyes may cause discoloration and greying of other laundry items. A bleaching system based on peroxide and an oxidation catalyst is one approach to inhibit dye transfer.
However, for a catalyst to be very effective in DTI, there are a number of requirements to be met [21]. The catalytic oxidation reaction should be fast, i.e. faster than the adsorption of textile dye to the acceptor fabric. If the fugitive dye is already adsorbed to the acceptor fabric it is much more difficult to bleach, since the reaction is now heterogeneous. Especially dyestuffs of the class of direct dyes adsorb very quickly onto cotton and only the most active catalytic systems can avoid dye transfer in this case.
As already outlined above for bleach catalysts in general, the stability of the catalyst is of importance. Textiles may loose textile dyes during the entire wash cycle, which can last up to 1 h, and the catalyst should hence be stable for at least this period of time.
In principle, a good catalytic system should be able to bleach a broad range of textile dyes, with strongly varying chemical structures. In practice, direct dyes for cotton exhaust dyeing, irrespective of the nature of the chromophore, will cause the most problems in dye transfer, since these dyes are not chemically bound to the fiber.
If a catalytic system is so efficient that the above requirements are met, there is the danger of unacceptable oxidative dye damage of the dyes on the fibers. While such dye damage may not occur after a single wash, it may occur after several wash cycles (cf. below) [29].
Below we will discuss some classes of oxidation catalysts studied by our own group, which have been proposed for use as DTI catalysts.
2.2 Manganese catalysts with phenolic ligands
Examples of this type of DTI catalysts include salen-, saltren- and bishydroxyphenyl triazole ligands complexed with Mn as shown in Fig. 1.
Mn complexes of salen ligands are well known as asymmetric epoxidation catalysts [30]. We found that certain Mn–salen complexes are active in oxidizing textile dyes in solution while the corresponding Fe catalysts are much less active [20,21,23,31,32]. The salen structure can easily be functionalized by applying substituted salicylaldehydes and/or various diamines. Relationships between the salen ligand structure and the DTI activity of the Mn(III)-complex have been published [31]. It was found that especially ligands with electron-donating substituents like hydroxy and dialkylamine in 4-positions of the aromatic rings are active catalysts compared to the unsubstituted salen complex. This might be related to the higher hydrolytic stability of the substituted complexes in alkaline aqueous solutions (results not shown). Moreover, the substitution alters the redox behaviour of the complex, making the Mn(III) center more easily reducible, as was confirmed by cyclic voltammetry (results not shown).
Whether or not these considerations play a decisive role for the explanation of the high DTI activity of the 4-substituted Mn–salen complexes is not clear, since for example the 4,4′-dimethyl amino substituted Mn–salen complex (1 in Fig. 2) is prone to a fast oxidative degradation in presence of hydrogen peroxide. In practice this means that such a Mn–salen catalyst shows a high catalytic activity in dye bleaching, but only for the first few minutes of a wash cycle [21].
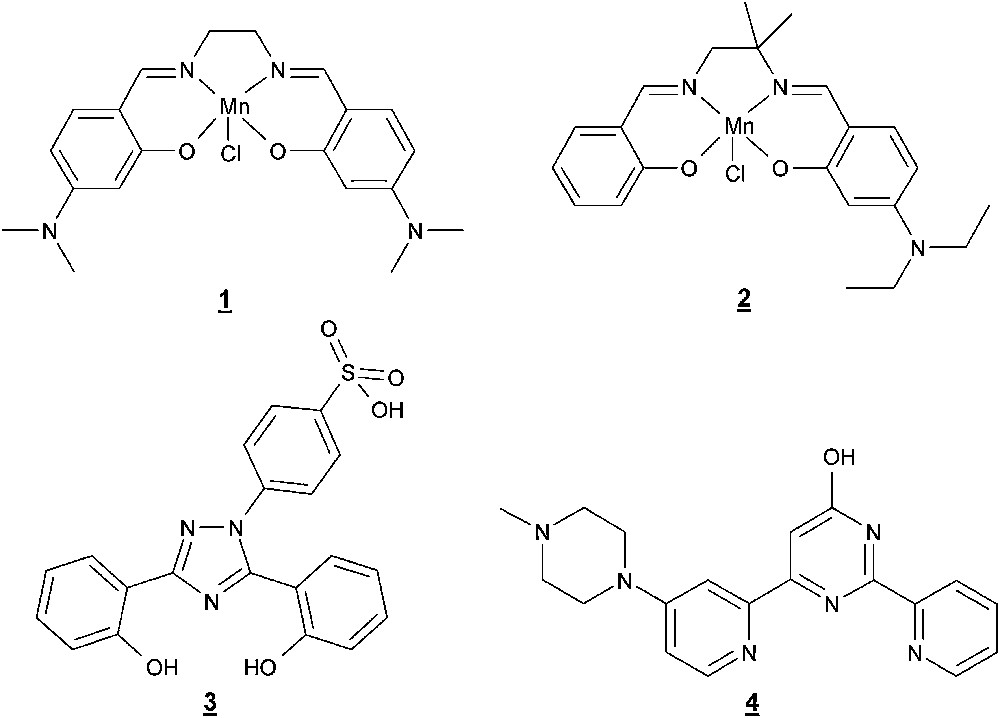
Selected Mn complexes and ligands of DTI catalysts.
This is illustrated in Fig. 3. The curve ‘without catalyst’ is a ‘dye bleeding curve’ and shows the typical rate by which a dye like R Bk 005 is released into the wash liquor (structure of R Bk 005 – see Fig. 5). Some dye is released quickly just in the beginning of the wash while a much slower release continues during the rest of the cycle. Upon addition of 50 μM of 1, the dye dosed to the system is bleached rapidly, but after about 3 min no more dye is bleached because no active catalyst is present anymore and at the end of the 30 min cycle only about 50% of the dye offered is bleached. Thus, despite the relatively high activity of this catalyst resulting in fast bleaching, the low stability does not lead to satisfactory results in practice [21].
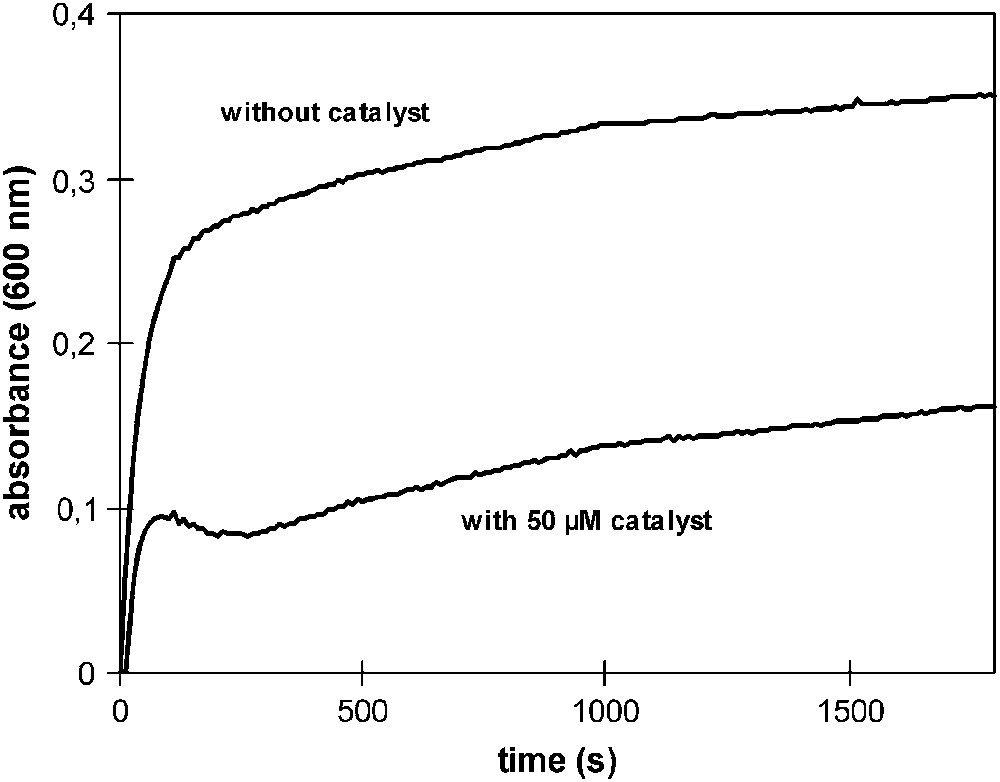
Effect of the unstable DTI-catalyst 1 on the bleaching of R Bk 005. Dye bleaching was studied in buffer at pH 10 and the absorption was measured at 600 nm. The curve ‘without catalyst’ is a typical ‘dye bleeding curve’ of a textile dyed with R Bk 005. The presence of catalyst significantly reduces absorption but only within the first few minutes.
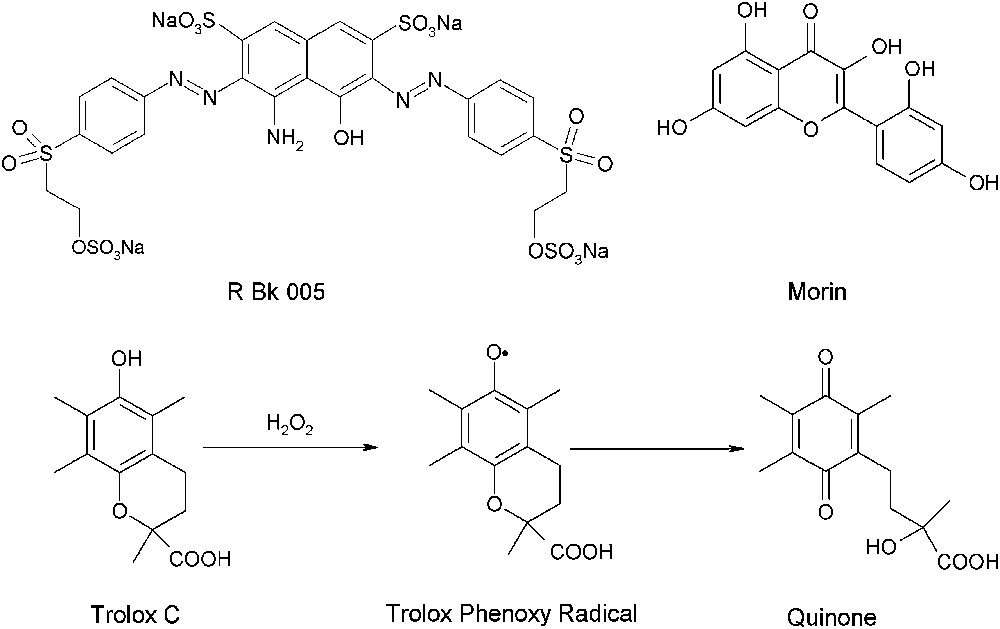
Typical model substrates used for the study of bleach catalysts. The figure contains also the degradation pathway of Trolox C.
Although a limited oxidative stability is characteristic for almost all Mn–salen complexes, we were able to identify an analog with a significantly enhanced oxidative stability. The Mn complex of the unsymmetrical salen ligand (2 in Fig. 2) was found to be one order of magnitude longer stable than 1 [21,33]. As a result, in a similar experiment as the one shown in Fig. 3, 95% of all added dye was bleached, since the complex was stable over the application time of 30 min [21]. An explanation in terms of the differences in chemical structure is far from obvious.
Unlike for the series of Mn–salen complexes, we found that in a series of substituted Mn(III)–saltren (Fig. 1) complexes the unsubstituted Mn–saltren complex was among the most active DTI catalysts [23,34]. This complex is about 6-fold faster in the bleaching of the dye R Bk 005 than the dimethylamino-substituted Mn–salen complex 1 (pH 10, 10 μM catalyst and 8.6 mM H2O2) and has recently been commercialized under the trade name TINOCAT© TRS. Considering the structure of this complex in the crystalline state it seems peculiar to observe any catalytic activity at all, since in crystals the Mn(III) center is coordinatively saturated by the three imine nitrogens and the three phenolic oxygens [35]. Most likely one or more of the coordinating O or N are detached in alkaline solutions in presence of H2O2 so that the Mn can interact with H2O2 or its anion.
A further class of Mn-catalysts with phenolic ligands is bishydroxyphenyl triazole type ligands (Fig. 1). One member of this type of ligands (3 in Fig. 2), is a very strong chelator for iron and is in use as a drug for treating chronic iron overload [36]. The Mn complex of 3 is an active DTI catalyst [24]. As for the salen ligands, the corresponding Fe complexes are not DTI-active. Overall the performance of these Mn–triazole catalysts is somewhat deficient to that of Mn–saltren.
For many Mn complexes of phenolic ligands the oxidative stability is a major drawback for obtaining satisfactory dye oxidation over the entire application time. A possible strategy to improve this is a slow release of a catalyst into solution from a solid “reservoir”, in which the catalyst is stable. In this case, fresh catalyst would be supplied over the entire wash cycle. Such a reservoir can be a slowly dissolving catalyst granule, for which the release rate can be tuned by the choice of the granule materials. It has recently been shown that this strategy can markedly improve the DTI performance [20,21].
A similar type of improvement can be achieved with Mn–saltren without granulation but with slowly dissolving crystals of the pure compound [23]. Mn–saltren is rather hydrophobic and dissolves only slowly in water. In this case the rate of dissolution can be tuned by the size of the complex crystals. While 20 μm crystals dissolve too slowly and do not give the desired bleach activity, milled crystals of about 1–2 μm dissolve quickly enough to provide a high DTI performance over the whole application time.
2.3 Bispyridyl pyrimidine complexes
Another group of highly active DTI catalysts are Fe(II) complexes with bispyridyl pyrimidine ligands (1:1 complexes) (Fig. 1) [25]. These complexes not only show an outstanding activity in oxidizing quite different textile dyes in solution but also show a high oxidative stability. Hence, the catalyst added at the beginning of the application remains active over the whole application time and a retarded release is not necessary.
For this type of ligands it is especially instructive to compare the different activities of Fe and Mn complexes. Fig. 4 compares the activity of 4–MnCl2 and 4–FeCl2 in oxidizing the textile dye R Bk 005 in solution (structure of 4 – see Fig. 2). The Fe(II) complex shows a distinctly higher activity than the corresponding Mn(II) complex. This holds not only true for this specific textile dye but also for many structurally quite different dyes. The reactivities of Fe and Mn complexes are very different on Morin (3,5,7,2′,4′-pentahydroxyflavone). Morin belongs to the flavonoid plant dyes and has hence a similar chromophore as many bleachable polyphenolic stains (Fig. 5). Since Morin is soluble under alkaline aqueous conditions it is frequently used as a soluble model substrate for a polyphenolic stain [37,38]. 4–MnCl2 shows a distinctly higher activity than 4–FeCl2 in bleaching Morin. This also correlates with the properties in the laundry application. While bispyridyl pyrimidine Fe complexes show a high activity in inhibiting dye transfer, the corresponding Mn complexes show a good activity in stain bleaching.
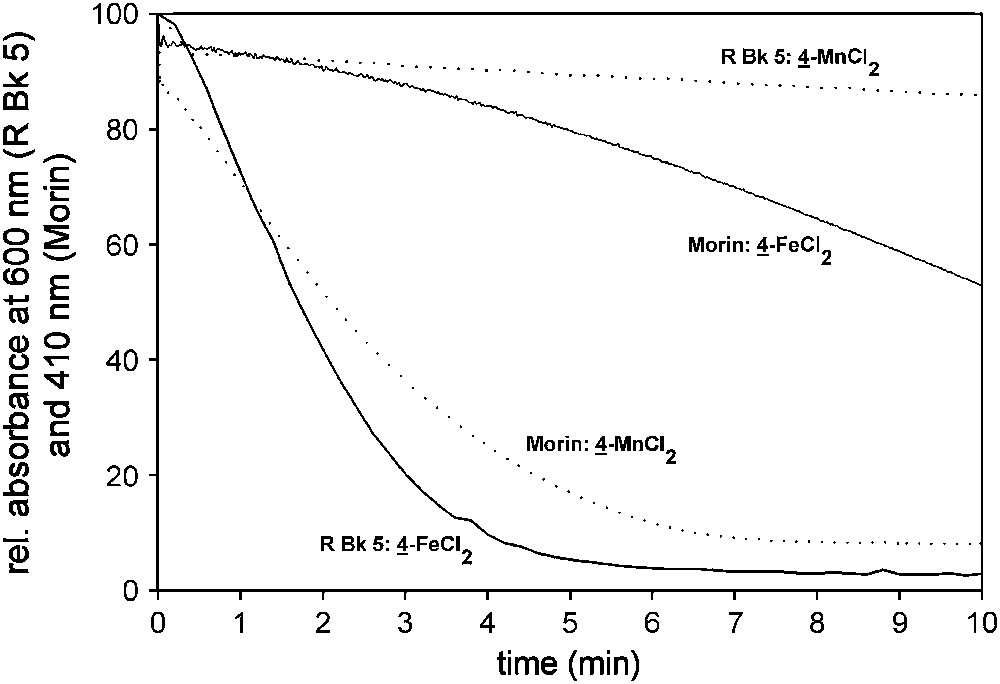
Bleach of Morin and R Bk 005 by 4–MnCl2 and 4–FeCl2. Morin bleach experiments were done in a cuvette containing 160 μM Morin, 10 μM catalyst and 10 mM H2O2. Bleach of R Bk 005 was done in solution containing 18.8 μM dye, 10 μM catalyst and 10 mM H2O2. Both experiments were done in 10 mM carbonate buffer (pH 10.0) at 23 °C.
The mechanism of the catalytic oxidation of Morin and R Bk 005 by H2O2 is widely unknown. A compound with a more defined degradation pathway is the water-soluble vitamin E analog, Trolox C (Fig. 5) [39]. Trolox C is known from the literature to be oxidized by a sequence of two one-electron oxidation steps [40]. The primary oxidation product of Trolox C is a sterically hindered phenoxy radical with a comparably long lifetime. A second one-electron oxidation step includes the disproportionation of two phenoxy radicals to Trolox C and the oxidation product 1,4-benzoquinone as the final product [39,40].
The formation and decay of the intermediate phenoxy radical can conveniently be followed by UV/vis spectroscopy because of its characteristic absorbance at 435 nm [13,40]. The quinone has a characteristic wavelength at 260 nm [13]. Fig. 6A shows the evolution of the phenoxy radical and Fig. 6B the formation of the quinone reaction product. Obviously, the concentration of the phenoxy radical is much higher for 4–FeCl2 than for 4–MnCl2 and also the formation of the reaction product is much faster. This illustrates a higher activity of the Fe(II) complex in oxidizing Trolox C by two one-electron steps than the Mn(II) complex. Hence, one can hypothesize that the high DTI activity of Fe(II) bispyridyl pyrimidine complexes might be caused by their ability to oxidize substrates by one-electron processes.
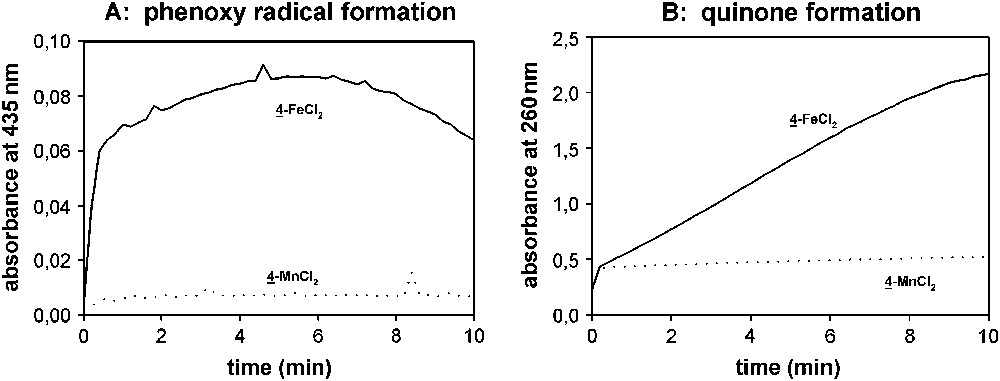
Trolox bleach with 2.5 μM 4–MnCl2 and 4–FeCl2, respectively, 300 μM Trolox C and 10 mM H2O2 in 10 mM carbonate buffer, pH 10.0 at 23 °C. (A) UV/vis traces at 435 nm indicating the build-up of the phenoxy radical. (B) UV/vis traces at 260 nm indicating the formation of the quinone reaction product.
3 Terpyridine–manganese complexes for stain bleaching
3.1 General catalytic properties of terpyridine–manganese complexes
Transition metal complexes of 2,2′:6′,2″ terpyridines are well known already for some time [41–44]. The potential use of terpyridine manganese complexes as oxidation catalysts has emerged only recently. A bis-μ-oxo-bridged Mn dimer of unsubstituted terpyridine [Mn2III,IVO2(terpy)2(H2O)2]3+ was synthesized in order to mimic the active center of the oxygen evolving complex of photosystem II [45,46]. The complex was shown to catalyze O2 evolution from hypochlorite and peroxy-monosulfate in water [45,47]. It is still a matter of debate, whether this complex is able to oxidize water or if the source of the evolving oxygen is the oxidant itself [48]. We have recently shown that π-donor substituted terpyridine–Mn complexes are able to effectively catalyze the oxidation of substrates by hydrogen peroxide under alkaline conditions (Fig. 7) [26]. They have also been shown to be effective stain-bleaching catalysts [27,28]. We review herein some of the typical features of this class of oxidation catalysts.
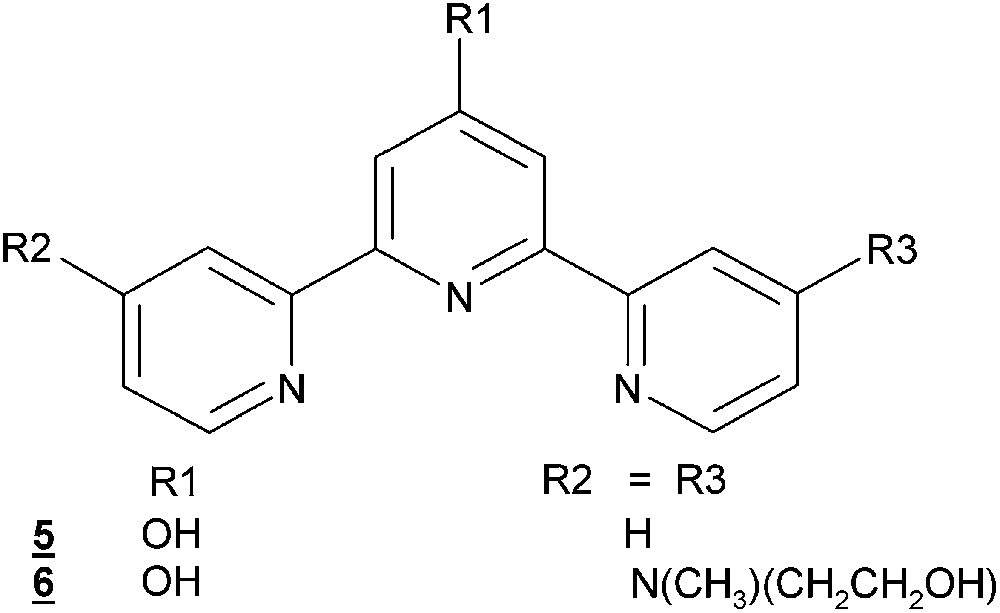
Substituted terpyridine ligands.
Terpyridine ligands are well known to readily form complexes with Mn(II) in solution. Depending on the stoichiometry, 1:1 or 2:1 (ligand to Mn) complexes may be formed [42,44,49]. Assuming that the existence of “free” coordination sites is of importance for a functional catalyst, 1:1 complexes are of highest interest for catalysis. A 1:1 stoichiometry also allows the formation of bis-μ-oxo-bridged dimers, thereby stabilizing Mn in higher oxidation states. In water, the complex formation constants of Mn(II)–terpyridine are not very high as expressed by log βML and log βML2 of 5.1 and 9.2, respectively [49]. Introduction of a hydroxy group in the 4′-position to give 5 (Fig. 7) considerably enhances the complex formation constants with Mn(II) to log βML = 7.9 and log βML2 = 13.9, i.e. by about two orders of magnitude for each complexation step. Therefore, introduction of electron pushing substituents into the 4′-position of terpyridine enhances the thermodynamic complex stability.
Substituted terpyridine–Mn complexes show a very good activity in bleaching polyphenols and carotenoids. Morin was again used as a soluble model substrate for a polyphenolic stain.
Fig. 8 shows the Morin bleach effect caused by 6–MnCl2 in presence of H2O2 at 23 °C as expressed by the decrease in the characteristic absorbance at 410 nm with time. 6–MnCl2 is extremely efficient in catalyzing the oxidation of Morin at pH 10 compared to MnCl2 and the non-substituted Mn–terpyridine 1:1 complex.
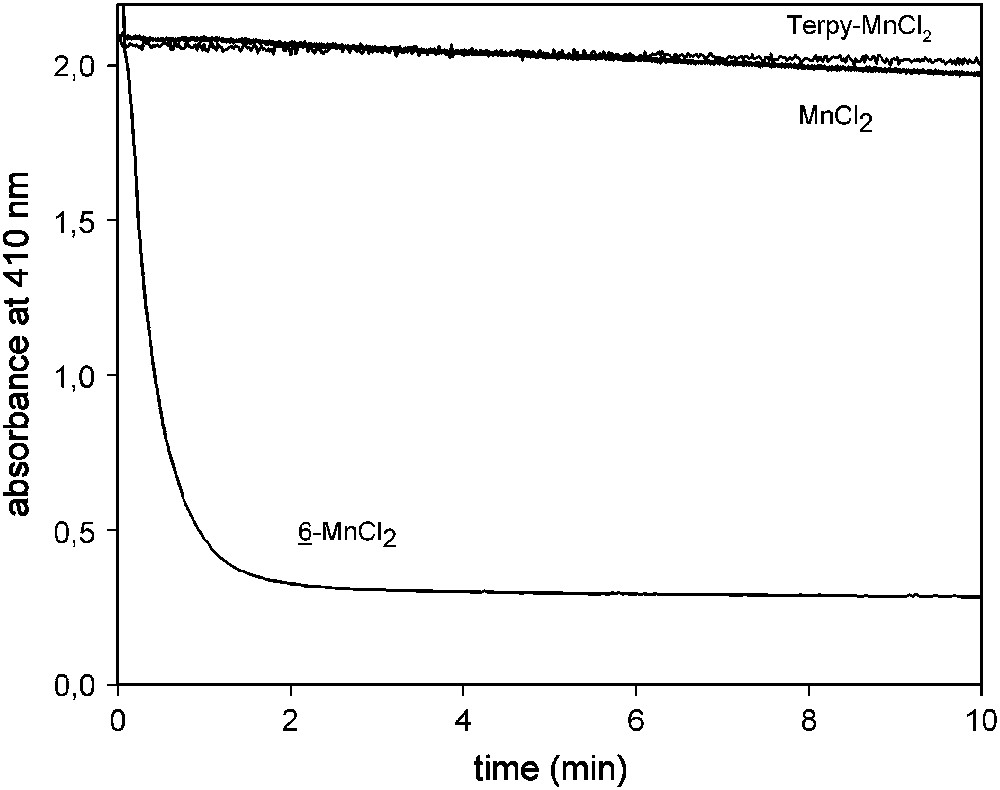
Morin bleach with 2.5 μM 6–MnCl2, MnCl2, and terpy–MnCl2 at 23 °C. Experiments were done in a cuvette containing 160 μM Morin and 10 mM H2O2 in 10 mM carbonate buffer at pH 10.
It should be noted that terpyridine–Mn complexes generally show a higher catalytic activity in Morin oxidation than the corresponding (same substitution pattern) bispyridyl pyrimidine–Mn complexes (see Section 2.3.)
The pH dependence of Morin bleach is illustrated in Fig. 9.
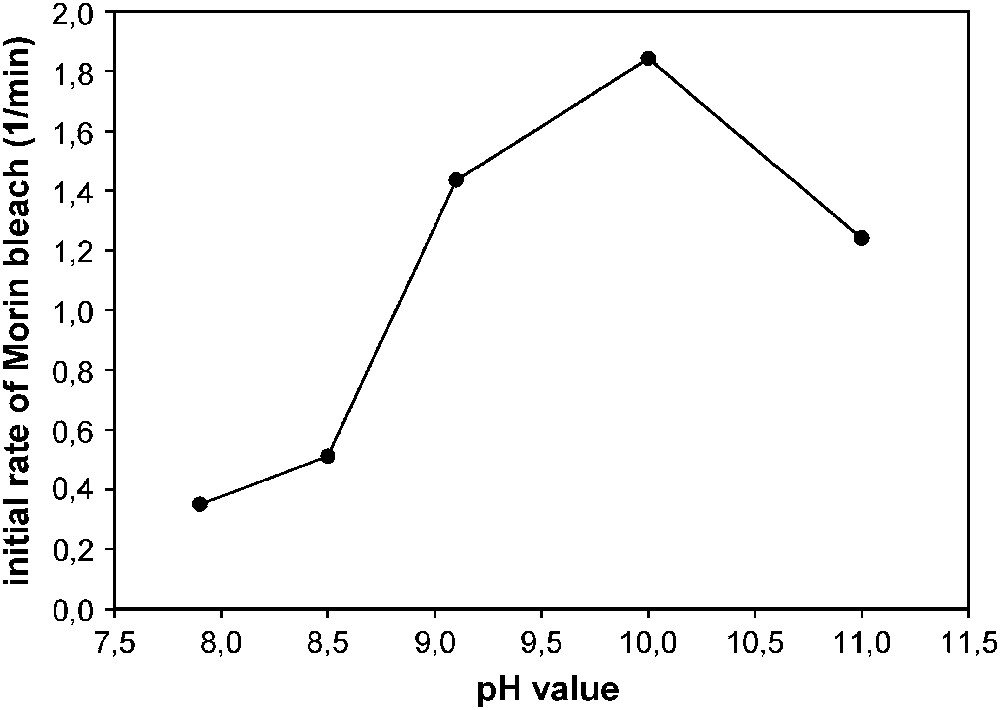
pH dependence of Morin bleach catalyzed by 2.5 μM 6–MnCl2, as expressed by the apparent initial Morin bleach rate. Experiments were done in a cuvette containing 160 μM Morin and 10 mM H2O2 at 23 °C. The pH was adjusted by 10 mM carbonate buffer (11, 10, 9.1) and 10 mM phosphate buffer (8.5, 7.9), respectively.
Lowering the pH of the system actually results in a dramatic drop in activity. The catalyst does not show any activity at acidic pH (not shown). Increasing the pH from 10 to 11 also reduces bleach activity. Interpretation of these results is not straightforward. Activity is generally influenced by the protonation state of the substrate, the catalyst, H2O2 and the solvent. Assuming that coordination of the perhydroxy anion to the complex is the initial step of peroxide activation, catalytic oxidation is expected to be favored by a high perhydroxy anion concentration, i.e. by a high pH. Moreover, Morin has pKa values of 3.5 and 8.1. Morin protonation might in part account for the low activity at pH values below 9. On the other hand, at very large pH values a complex may be destroyed by the formation of Mn(hydr)oxides. This could account for the drop in activity observed at pH 11.
The assumption that a 1:1 complex is the catalytically active species is reinforced by the fact that the catalytic activity of a 1:1 complex can be drastically reduced by adding an excess of ligand, i.e. by promoting the formation of the 2:1 complex (not shown). A 2:1 complex is coordinatively saturated and hence catalytically inactive.
Besides a sufficient activity, effective bleach catalysts must show a sufficient functional lifetime. With respect to the laundry application, a lifetime between 30 and 60 min is advantageous. This is typically the case for many terpyridine–Mn complexes in presence of hydrogen peroxide. Experiments with multiple additions of Morin as a substrate revealed that 6–MnCl2 only slightly loses activity within the timeframe of the experiment, thereby proving an extremely high oxidative stability of the ligand system [27].
The non-productive disproportionation of H2O2 (catalase) is a general property of Mn complexes possessing catalytic activity towards organic substrates. Terpyridine–Mn complexes have been shown previously to effectively degrade hydrogen peroxide [26]. This is normally unwanted and generally limits the cost effectiveness of a bleach system. An optimization of the bleach system with respect to a high bleach effect combined with an acceptable rate of peroxide decomposition is hence often necessary. With regard to the laundry bleach process, many terpyridine–Mn complexes bleach well with the peroxide level typically present in today's European detergents.
Although hydrogen peroxide is an environmentally benign and comparably inexpensive oxidant for bleach processes, the ultimate oxidant of choice is oxygen from the air. The use of oxygen from the air in laundry would also reduce the chemical loading, since peroxide is currently provided from persalts, which can assume up to 20% of a typical detergent formulation. Manganese–terpyridines are to a certain extent also able to activate molecular oxygen [50]. Fig. 10 illustrates the ability of 6–MnCl2 to catalyze the oxidation of Morin in air-saturated alkaline solution compared to the non-catalytic peroxide system and MnCl2. The catalyst/air system shows a distinctly higher activity than hydrogen peroxide only. It should, however, be noted that the overall activity is lower than that of the peroxide/catalyst system (cf. Fig. 8; note that a higher catalyst concentration was used for air bleaching and that the experiment was done at higher temperature!).
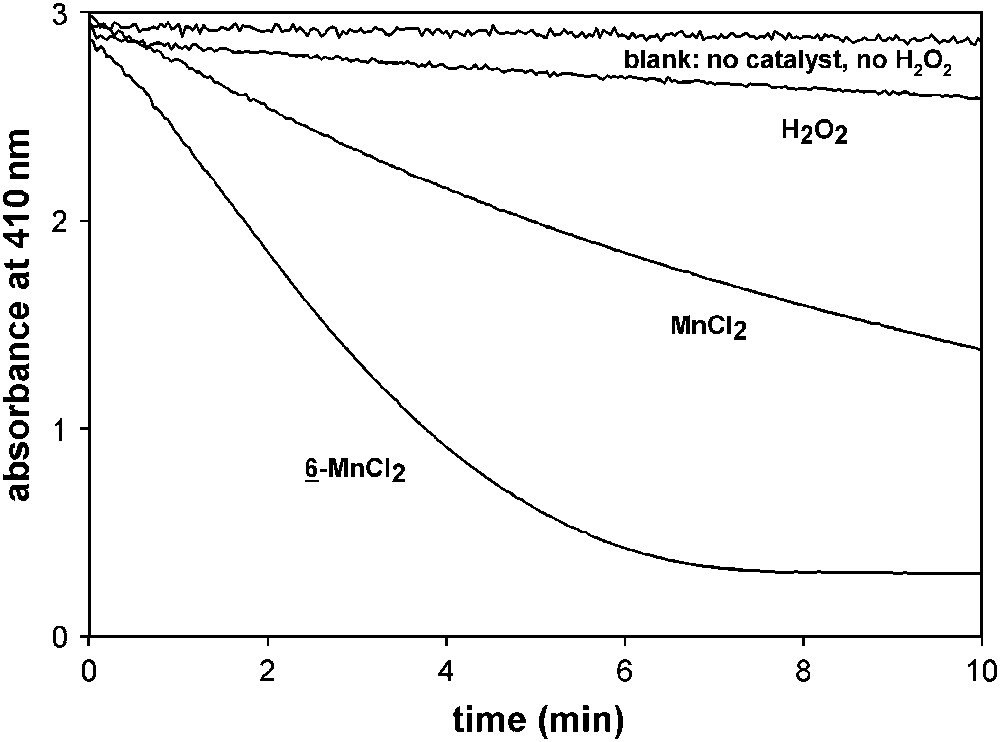
Morin bleach by oxygen of an air-saturated solution containing 10 μM 6–MnCl2 and MnCl2, respectively, at 40 °C. Experiments were done in a cuvette containing 160 μM Morin in 10 mM carbonate buffer at pH 10. For comparison, the figure also contains data of Morin bleach by 10 mM H2O2.
Although only exemplified for 6–MnCl2 herein, it should be noted that the catalytic features described above are typical for many substituted terpyridine–Mn complexes.
3.2 Optimization of terpyridine structure for bleach activity
The results with 4-substituted terpyridines shown above have already suggested that the catalytic activity of the Mn(II) complexes in presence of hydrogen peroxide is greatly enhanced by the presence of π-electron donor groups. Quantitative structure–activity relationships represent an attempt to correlate structural descriptors of compounds with activities [51]. A simple approach, frequently used for aromatic systems is the Hammett equation, which is widely applied in the form:
Here, a linear free energy relationship of a set of terpyridine manganese(II) (1:1) complexes was obtained from kinetic data on the oxidation of Morin in a similar experiment as shown in Fig. 8. The catalysts investigated in the present study are summarized in Table 1.
Initial rate of Morin bleach and substituent constants of terpyridine ligands
Substituent 4′ | Substituents 4 (5), 4″ (5″) | Σσ | Initial rate k (1/min) | Substituent 4′ | Substituents 4 (5), 4″ (5″) | Σσ | Initial rate k (1/min) | ||
OH | −2.33 | 3.78 | OEt | OMe | OMe | −0.75 | 0.06 | ||
−2.28 | 3.66 | H | H | −0.40 | 0.10 | ||||
OH | −1.81 | 2.83 | H | 5: | 5″: | −0.32 | 0.07 | ||
NH2 | NH2 | ||||||||
OH | −1.61 | 0.39 | OEt | H | H | −0.24 | 0.07 | ||
−1.50 | 1.37 | H | H | H | 0.00 | 0.06 | |||
OH | H | −1.31 | 1.04 | H | 5: | 5″: | 0.16 | 0.04 | |
OH | H | −1.21 | 1.25 | H | 0.20 | 0.02 | |||
OMe | −1.20 | 0.82 | H | 0.20 | 0.01 | ||||
−1.07 | 0.08 | H | 5: | 5″: | 0.20 | 0.02 | |||
OEt | OEt | ||||||||
OEt | −0.88 | 0.08 | H | 5: | 5″: | 0.24 | 0.03 | ||
OMe | OMe | ||||||||
OH | H | H | −0.81 | 0.18 | H | 5: | 5″: | 0.42 | 0.01 |
OBu | OBu | ||||||||
H | H | −0.76 | 0.14 | H | 0.46 | 0.01 |
The compounds include unsubstituted 2,2′:6′,2″ terpyridine; 4′ (para-); 4,4′,4″ (para-) and 5,5″ (meta-) substituted derivatives. The apparent initial rates of Morin bleach k were calculated and are listed in Table 1.
The substitution constants of complexes were calculated by summing up the Hammett constants of each substituent on the pyridine rings of 2,2′:6′,2″ terpyridine [52]. By definition the substitution constant of 2,2′:6′,2″ terpyridine is zero.
A linear free energy relationship of log k/k0 versus Σσ was obtained with a slope of −0.91 (Fig. 11; r2 = 0.893).
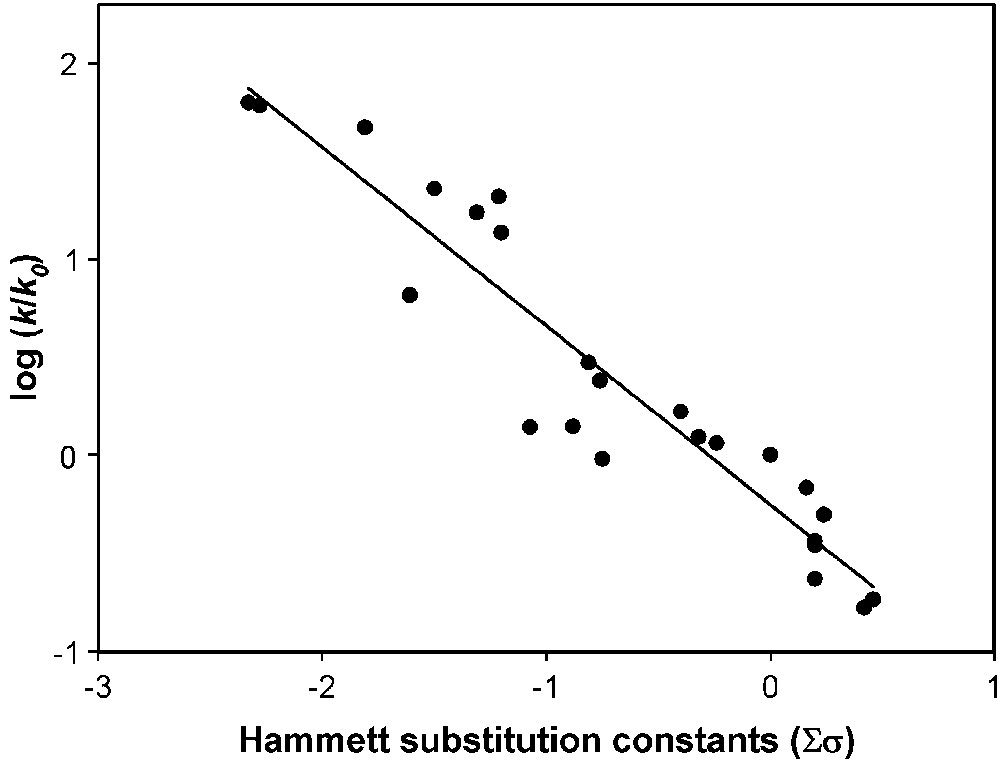
Hammett correlation (r2 = 0.893) of a set of terpyridine–Mn complexes in Morin bleaching. Morin bleach experiments were carried out in 10 mM carbonate buffer (pH 10) containing 160 μM Morin, 10 μM complex and 10 mM H2O2 at 23 °C.
The distinctly negative slope indicates that electron-donating substitutions in the 4-positions significantly promote catalytic activity. This might be caused by a stabilization of Mn in higher oxidation states due to the presence of electron-donating substituents. Furthermore, electron-donating substituents in the 4-positions increase the thermodynamic complex formation constant of Mn–terpyridine complexes (cf. above).
Although a correlation between log k/k0 and Σσ clearly exists, the data scatter considerably. Possible reasons for the scattering are manifold and could include, for example, different solubilities, differences in complex stability, different susceptibilities to side reactions such as catalase reaction or different steric effects. Furthermore, assuming an equal impact of the outer and inner pyridine rings is probably an oversimplification.
Unlike substituents in the 4-positions, electron-donating substituents in the 5- and 5″-positions result in the same or even a reduced catalytic activity compared to the non-substituted terpyridine. This is in line with the fact that the same substituents in meta position possess positive or near-zero substituent constants, indicating the absence of a noticeable electron-donating effect at the pyridine nitrogens.
3.3 Influence of complex oxidation state on the bleach effect
Terpyridine–manganese complexes may exist in different oxidation states. From the literature, stable 1:1 (ligand:manganese) complexes are known not only with Mn(II) centers [53] but also with Mn in a higher oxidation state, e.g. bis-μ-oxo-bridged Mn(III)–Mn(IV) complexes [45,46]. Functional studies with hypochlorite as oxidant suggested the participation of bis-μ-oxo-bridged species with manganese in oxidation states between III and V in the catalytic cycle of O2 generation [45].
We have synthesized a bis-μ-oxo-bridged Mn2(III, IV) dimer of ligand 5 following the synthesis procedure in the literature (Mn2(III, IV)(5)2O2) [28,45]. The antiferromagnetic coupling of the two Mn centers and their oxidation states were proven by the presence of the characteristic 16-line EPR spectrum in DMF/water (1:1) (Fig. 12). Addition of H2O2 to a solution of this complex at ambient temperature and freezing of the sample after 1 min directly resulted in the typical 6-line high-spin Mn(II) signal, revealing that hydrogen peroxide does not act as an oxidant but instead as a reductant under these conditions. Unfortunately, similar experiments with H2O2 were not possible to perform under alkaline conditions because of the paramagnetic disturbances arising from H2O2 disproportionation. UV/vis spectroscopy has instead been used to study the complex in presence of peroxide at pH 10. Fig. 12 shows Mn2(III, IV)(5)2O2 together with an in-situ complex formed between the same ligand and Mn(II). The shoulder above 400 nm for Mn2(III, IV)(5)2O2 is in accordance with the deep green color of the solid complex. The figure also shows both spectra after the addition of H2O2. Interestingly, both spectra are identical in presence of peroxide and resemble the spectrum of the Mn(II) complex. Therefore, these results suggest that also at pH 10, the Mn(III)–Mn(IV) complex is reduced to Mn(II) in presence of hydrogen peroxide.
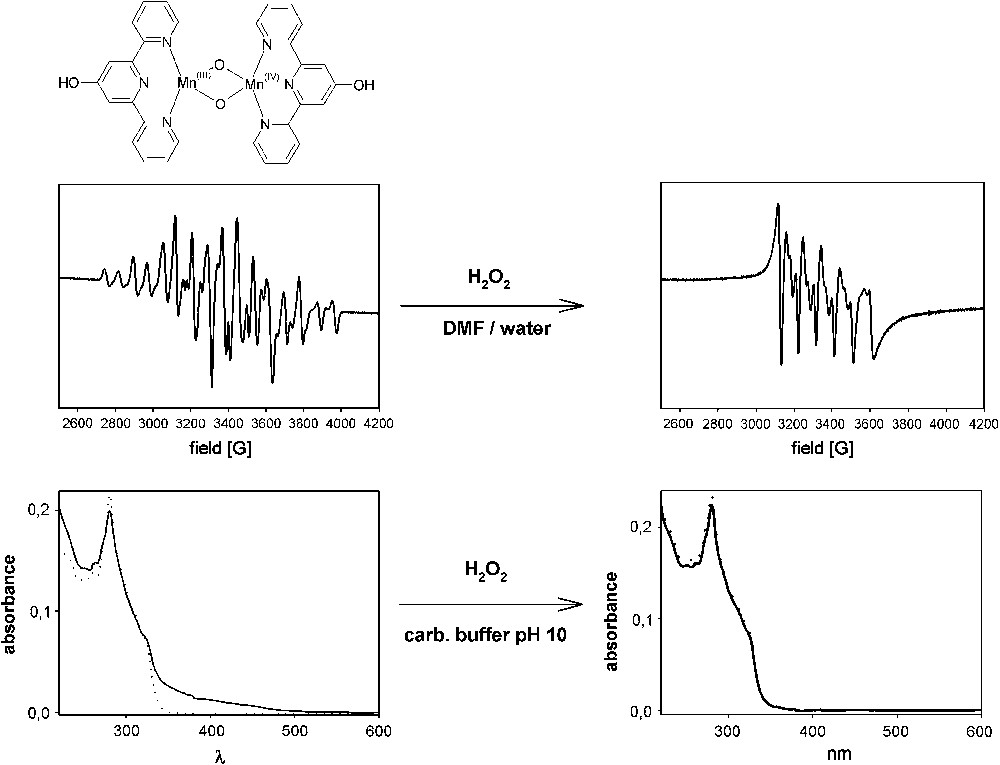
EPR and UV/vis spectroscopies of Mn2(III, IV)(5)2O2 in DMF/water and 10 mM carbonate buffer (pH 10), respectively. EPR measurements were done with a Bruker X-band EPR spectrometer at 77 K. EPR spectra of a 1 mM solution of Mn2(III, IV)(5)2O2 are shown before and after addition of 5 mM H2O2. Likewise, UV/vis spectra of 7.3 μM Mn2(III, IV)(5)2O2 (solid lines) are shown in 10 mM carbonate buffer (pH = 10) before and after addition of 1.1 mM H2O2. The figure also contains UV/vis spectra of 5–MnCl2 (dotted lines).
Stain-bleaching experiments with the Mn(II) complex of 5 and Mn2(III, IV)(5)2O2 revealed the same performance for both complexes (data not shown). Obviously, addition of peroxide reduces the Mn(III)–Mn(IV) complex to the Mn(II) complex, which is suggested to be the resting state in the catalytic cycle. Therefore, for the bleach application the oxidation state of the used catalyst precursor seems to be less important.
4 Side effects of catalytic bleach: dye and fiber damage
4.1 Dye damage of bleach catalysts
For a broad market introduction a bleach catalyst has to show not only a good performance in stain bleaching or dye transfer inhibition, but also at the same time it must not lead to premature dye fading or fiber damage. The number of different textile dyes in the market is huge and their chemistry is diverse. Of course, it is hardly feasible to find a catalyst with a broad stain bleaching profile and no damage at all on any textile dye. The decision on the dye safety has hence to be based on a risk assessment considering the performance benefits on the one hand and the damage profile on the other hand [54]. Several papers have described the mechanisms of dye bleaching in presence of transition metal complexes [55–58]. Typically, the oxidation mechanism is not only determined by the catalyst, the oxidant and the substrate, but also by the specific environment, i.e. the solvent and the pH. In solution experiments with Mn–Me3TACN (pH 10.5), Gilbert et al. reported that phenols are oxidized by an initial one-electron process from the phenolate ion to the phenoxy radical [13]. In another paper the same group studied the oxidation of different azo dyes by the same system [55]. No evidence for one-electron reduction was observed for the dimer [MnIV(μ-O)3MnIV(Me3TACN)2]2+. However, mononuclear Mn(III) Me3TACN species were suggested to catalyze dye oxidation via a catalytic cycle involving (i) a two-electron oxidation of Mn(III) species to Mn(V)-oxo by peroxide followed (ii) by two one-electron reductions of the complex by the dye anion.
A study of the bleach of azo dyes by hydrogen peroxide, catalyzed by manganese porphyrin derivatives, suggests the initial formation of a substrate–catalyst complex [56].
Hodges et al. [57] reported different mechanisms at different pH in the oxidation of azo dyes catalyzed by a sterically hindered anionic oxoiron(IV) porphyrin. In strongly alkaline solution, as used for laundry processes, the dominant reaction is electron transfer. However, at neutral pH the bleach proceeds via an initial hydrogen abstraction from the substrate. In another study radical formation on dyed cotton substrates as determined by EPR was correlated with dye and fiber damage for the Mn–Me3TACN/H2O2 system [58]. No correlation between the presence of free radicals and the extent of dye damage could be found.
As indicated above the mechanistic study of the catalytic oxidation of individual dyes gives only limited insight into the dye damage profile of a bleach catalyst and it is currently hardly feasible to predict dye damage based on mechanistic understanding. Several hundred different dyes are used for textile dyeing and it is hence necessary to check the dye safety on a limited selection of dyes. Different dye sets are used in the industry and there are attempts to harmonize the dye sets [29]. The selection of dyes typically considers the market relevance, the chemical diversity of dye classes, color preferences and the different dyeing technologies. A proper dye set gives a reasonable overview of the potential damage of a catalytic system. However, it cannot rule out that a catalyst may cause significant damage on a dye, which does not belong to the test set.
In the following we will illustrate how a dye set containing 30 different dyes is used to study the dye safety of catalysts. Dyed cotton fabrics were washed repeatedly under European washing conditions in presence of 1.5 μM Mn–Me3TACN and 6.1 μM 5–MnCl2, respectively. For comparison, we also studied the dye safety of 0.38 g/L of the activator TAED. A standard detergent was used containing sodium percarbonate as the oxidant. Under these conditions, 5–MnCl2 gives rise to the same bleach performance as the activator system. Mn–Me3TACN shows a better bleach performance than the TAED/percarbonate system. The reflectance spectra of the fabrics before and after washing were measured with a Spectraflash 2000 spectrophotometer. The change in color ΔE (ΔE = [(ab − aa)2 + (bb − ba)2 + (Lb − La)2]0.5) was calculated according to the CIE standard procedure after eight consecutive washing cycles (aa, ba, La) relative to the untreated fabric (ab, bb, Lb) [59].
Table 2 shows ΣΔE values, i.e. the sum of ΔE values over the 30 dyes tested, for each bleach system studied at 40 and 60 °C.
Dye damage caused by different catalysts compared to TAED
TAED | 5–MnCl2 | Mn–Me3TACN | |
ΣΔE, 40 °C | 62 | 35 | 67 |
ΣΔE, 60 °C | 100 | 50 | 111 |
ΔE, 60 °C R Bk 005 | 12.7 | 3.3 | 18.9 |
ΔE, 60 °C R Re 264 | 11.6 | 4.6 | 8.1 |
ΔE, 60 °C R Ye 205 | 2.0 | 0.5 | 0.9 |
ΔE, 60 °C R Bl 021 | 1.6 | 1.0 | 4.3 |
Catalyst 5–MnCl2 shows the lowest damage of textile dyes. The overall damage caused by Mn–Me3TACN is somewhat higher than that of TAED, however, not dramatically worse. To obtain a better understanding of the impact of dye damage it is necessary to look at specific dyes. The table shows also the results for four individual dyes of the dye set as an example. Mn–Me3TACN shows a higher damage than TAED on R Bk 005 and R Bl 021. However, the damage on R Ye 205 and R Re 264 is lower than that of TAED. Considering that R Bk 005 is the most important reactive dye on the market and that the damage of Mn–Me3TACN is strongly elevated on this dye, it must be concluded that the use of Mn–Me3TACN would not be acceptable under these application conditions in a laundry detergent.
4.2 Fiber damage of bleach catalysts
Cellulose based fibers mainly from cotton are still the most frequently used fibers in textiles. Oxidative damage of these fibers typically results in a decrease in the degree of polymerization. Macroscopically, this is expressed by a loss in the tensile strength. While the degree of polymerization is determined by viscosity measurements of a cotton solution, which is prepared in a specific solvent, the tensile strength loss is measured mechanically [60]. Transition metal induced fiber damage is known from industrial cellulose bleaching processes, which are typically carried out at high temperatures (90–100 °C) and high peroxide concentrations. Fiber damage was also reported for Mn–Me3TACN containing laundry detergents and was one of the reasons for their withdrawal from the market [2]. In both cases (industrial cellulose bleaching, Mn–Me3TACN in detergents) transition metal initiated OH/OOH radical formation was suggested to be responsible for the damage [61–64].
Although Arthur and Hinojosa [65] found evidence for cellulose radical formation with FeSO4 and H2O2 at 110 °C, the theory of OH/OOH radicals being responsible for catalytic fiber damage still lacks substantial scientific evidence. Since transition metal catalysts may lead to transition metal accumulation on cotton fibers after multiple washes (most likely as deposits of mineral forms or as ions complexed with partially oxidized glucose units), it was straightforward to assume that damage is caused by the accumulated transition metals. However, in a recent paper it was shown that enhanced Mn levels on fibers do not necessarily result in increased fiber damage [66].
This conclusion is reinforced by a simple multiple wash experiment. White cotton fabrics were washed 10 times under European washing conditions in presence of 4.5 μM MnCl2 or 2.25 μM Mn–Me3TACN (equivalent to 4.5 μM Mn in solution). The degree of polymerization of the cotton fibers before the washes was 1670. In presence of Mn–Me3TACN the degree of polymerization dropped significantly to 1030 after 10 washes, while in presence of MnCl2 the degree of polymerization was 1500 after the washes. The deposition of Mn on the fibers was found to be 6 ppm for the Mn–Me3TACN system and 5 ppm for the MnCl2 system. Obviously, although the amount of Mn deposition on the fiber was very similar, both bleach systems give rise to quite different damage. The severe damage caused by Mn–Me3TACN suggests that the damage is caused by reactive species generated in the course of the catalytic reaction rather than by the deposition of Mn on the fiber.
To date, our knowledge on catalyst induced damage of cellulose fiber is still very limited. A deeper understanding of mechanistic aspects of catalytic stain bleaching and fiber degradation could help to be able to uncouple both effects and to design highly effective but fiber-safe bleach systems.
Acknowledgement
The authors thank M.-J. Dubs, U. Heinz, F. Bachmann and J. Dannacher for their contributions to this work.