1 Introduction
Recently, there has been a growing interest in exploring transition metal complex catalysts immobilized on polymeric organic matrices. Polymer-supported transition metal complexes have started to be looked at as good alternatives to typical inorganic carriers. The main advantage of polymer supports is the chemical, physical and morphological structure of these materials and its influence on the catalytic properties of the metal complex immobilized on them. The use of epoxy resins as carriers of catalysts has been limited so far to the use of the metal complex as the polymerization initiator and the precursor of the catalytic centres in the cured resin [1,2] and to the application of various matrices functionalized with epoxy groups for the immobilization of proteins and enzymes to improve their biocatalytic properties [3]. Such resins were found to have a high capacity and selectivity towards metal-ion species. For example, a sulfur analogue of the glicidyl methacrylate resin was synthesized by Moore et al. [4], in which the oxirane group was replaced by a thiirane ring. The ring-opening reaction of the thiirane group with amines or other nucleophiles generates a thiol group at the β-carbon atom. Such immobilization of ligands produces ion-exchange resins that may be used in wastewater treatment and in the regeneration of metal salts in industrial processes. In these ion-exchange resins, not only the metal binds to the ligand, but also the thiol group takes part in metal-ion binding, thereby acting as additional donor site. Crudden et al. showed that thiol-functionalized mesoporous silicates are efficient Pd scavengers and that the resulting Pd-encapsulated materials catalyze the Suzuki–Miyaura and Mizoroki–Heck coupling reactions with virtually no leaching of Pd. Several heterogeneity tests were performed on the catalyst under Suzuki–Miyaura conditions and indicate that the main part of the catalysis (> 95%) occurs on the support via a truly heterogeneous catalyst. Studies are ongoing to determine the structure of the catalyst and the scope of its activity [5]. Magnetic resins with amine and mercaptan as chelating groups were prepared by suspended condensation polymerization of 2-chloroethoxymethyl thiirane with diamines. The resins show a high affinity for noble-metal ions and Hg(II), and predominantly adsorbed Pd(II) or Hg(II) with Cu(II), Zn(II), and Mg(II) coexisting [6].
In our earlier works, we proposed a similar approach and used thiol-functionalized epoxy resins as coatings for magnetic cores in encapsulation processes. This novel type of supports for metal complex catalysts can be easily separated by the use of a magnetic field. Our experiments have shown that the microporous structure of the polymer supports in the hydrogenation of cinnamaldehyde seems to have higher impact on the reaction activity and selectivity as compared with the influence of the chemical structure of the supports. The morphology parameters, such as pore-size distribution and specific surface area depends on the type of thiol used as resin hardener [7].
In recent years, scCO2 had many applications in many fields of chemistry. Supercritical fluids have a unique and valuable potential for the enhanced processing of many materials. The applications of supercritical fluids to polymer processing are known. The ability of supercritical carbon dioxide to swell and plasticize polymers is crucial to the impregnation, extraction, and modification of polymeric materials [8]. Supercritical fluid dyeing technology has received attention in the textile industry due to increasing environmental concerns. The solubilities of three novel disperse azo dyes in supercritical carbon dioxide were measured. The results show that this method is very promising for the development of supercritical processes for dyeing applications based on these dispersed dyes [9]. A thin film of polymer was fabricated on a functionalized silicon wafer through self-assembled monolayers (SAMs) of perfluorophenyl azide derivatives (PFPA-silane) with covalent bonds by photochemical reaction. The SAMs were formed in supercritical carbon dioxide and the immobilization of polymers was performed by UV irradiation. The results indicate that scCO2 is a good solvent for silylation reactions, better than common organic solvents such as toluene [10]. Supercritical fluids allow the synthesis of many types of particles since the solvent's chemical and physical properties can be varied with temperature or pressure, both of which can affect the degree of supersaturation and nucleation. Hakuata reported methods for the formation of fine particles using supercritical fluids CO2 and water [11]. Carbon dioxide in its liquid or supercritical state (scCO2) has a prodigious potential as an environmentally benign reaction medium for sustainable chemical synthesis. Since the mid 1990s, rapidly increasing research efforts have shown that scCO2 can replace conventional and potentially hazardous solvents in a wide range of processes. There is also increasing evidence that the application of scCO2 can broaden the scope of catalytic synthetic methodologies [12].
Herein, we reported the synthesis of some efficient heterogenized palladium catalysts based on supports prepared from epoxy resin cured with thiol-terminated polythiourethane. These novel effective curing agents were synthesized from low-molecular-weight di- and multifunctional mercaptans and diisocyanates [13]. The oligomeric polythiourethanes, apart from their good curing characteristic of epoxides, allow one to introduce new binding ligands into the structure of polymers. The immobilization of the homogeneous catalyst was carried out under supercritical conditions. The use of supercritical CO2 will enable a more accurate and better distribution of the metal complex in a polymer matrix. The activity of these new catalysts was checked in the hydrogenation reaction.
2 Experimental
2.1 Materials
As epoxy resin, a high-purity bisphenol A diglycidylether D.E.R.™332 (DER), epoxy equivalent 170 (viscosity 4000–6000 mPa·s at 25 °C), The Dow Chemical Company, USA, was used. A multifunctional polythiourethane hardener (MPTU) was prepared by the reaction of hexamethylene diisocyanate (HDI) and pentaerythritol tetrakis (3-mercaptopropionate) (SIGMA) in excess molar quantity (by 2 moles) using a previously reported method [13]. Homogeneous catalyst PdCl2(PhCN)2 was prepared from PdCl2 (Sigma, 99%) and benzonitrile (Sigma, 99%) in petroleum ether (Sigma) [14]. Toluene (POCH), trans-cinnamylaldehyde (Aldrich), ethanol (POCH) were used for the hydrogenation reaction, without further purification.
2.2 Support preparation
Three grams of epoxy resin (DER) and 1.2 g of polythiourethane (MPTU) were placed in a flask and mixed until a homogenous consistency was obtained. The mixture was transferred into a Teflon® mold and cured at temperatures from 298 to 423 K over a time period of 1 h to 48 h. The cured resin was frozen by immersion in liquid nitrogen and mechanically ground to powder. The particle sizes were about 0.5 mm, which was determined by sieve analysis.
2.2.1 Preparation of the catalyst
On the prepared support, we immobilized the palladium complex PdCl2(PhCN)2 from a saturated toluene solution through a ligand-exchange process under supercritical conditions. The supercritical immobilization set-up is represented in Fig. 1. It is mainly composed of a 100 ml stainless steel autoclave equipped with a thermostat, a temperature controller and a pressure indicator. In a typical experiment, 0.5 g of the support and 15 ml of a saturated solution of the palladium complex were introduced into the glass tube, which was placed at the bottom of the autoclave. The autoclave was heated to the set temperature and filled with liquid CO2 through a high-pressure pump until the desired pressure has been reached. The immobilization was carried out for 5 h at 300 K and at a pressure of 90 bar. The supercritical phase, containing the catalyst precursor, diffuses inside the polymer matrix during a pre-established impregnation duration. At the end of this period, CO2 is vented out of the cell through the depressurization valve in isothermal conditions. For comparison, the immobilization process was also carried out by a standard immobilization procedure. The mixture of the same amount of Pd complex solution and support was stirred at room temperature for 48 h. The products of both processes were filtered off, washed with toluene (3 × 20 ml) to remove the quantity of complex that was non-chemically bounded to the polymer and finally dried under vacuum at 50 °C for 8 h.
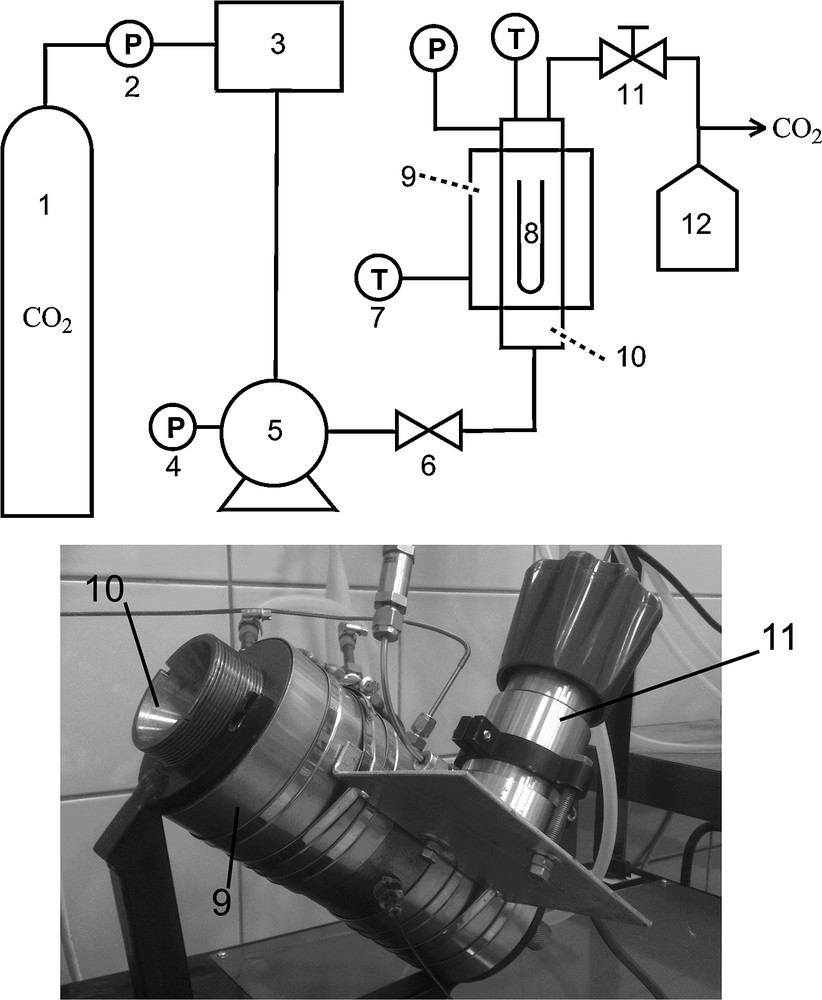
Scheme of supercritical immobilization equipment; photograph of the autoclave with the thermostated bath: (1) CO2 bottle; (2, 4) pressure gauge; (3) cooling bath cryostat; (5) liquid pump; (6) needle valve; (7) temperature sensor; (8) glass tube; (9) thermostated bath; (10) autoclave; (11) pressure reduction valve; (12) solvent trap.
2.3 Spectroscopy characterization
The FT-IR spectra were measured with a BIORAD FT-IR 175 C spectrophotometer under an air atmosphere. XPS measurements were made on a VG ESCALAB 210 spectrometer with a Mg Kα (hν = 1253.6 eV) excitation from an X-ray tube (reduced power 10 kV, 10 mA). The pressure in the spectrometer chamber was about 10–9 mbar. The samples were pressed to pellets under a pressure of 100 kbar for 10 min before these measurements. The C 1s, N 1s, O 1 s, Cl 2p and Pd 3d core level spectra were recorded. The analyzer pass energy was set at 20 eV. A take-off angle of 90° was used in all XPS studies. Curve fitting was performed using the ECLIPSE data system software. This software describes each of the components of a complex envelope as a Gaussian–Lorentzian sum function. The background was fitted using a non-linear model function proportional to the integral of the elastically scattered electrons. All binding energies (BEs) were referenced to the C 1s neutral carbon peak at 284.6 eV.
2.4 Study of Pd leaching
ToF–SIMS measurements were performed using an ION-ToF GmbH instrument (ToF-SIMS IV) equipped with a 25-keV pulsed Bi+ primary ion gun in the static mode (primary ion dose of about 1.5·1011 ions/cm2). The analyzed area corresponds to a square of 500 × 500 mm in the case of secondary-ion mass spectra collecting and surface imaging. For each sample, three spectra from different surface areas were recorded. To obtain the plain surface of catalysts (then better mass resolution could be achieved), powder samples were tableted before the measurements. In order to compare the quantity of palladium present on the surface of the catalysts before and after the reaction, the number of counts of the selected ions obtained from the collected mass spectra was normalized on the basis of the number of total counts.
2.5 Morphology studies
The morphology of the samples was investigated with a scanning electron microscope JEOL 5500 LV, working under high vacuum and with an accelerating voltage of 10 kV. An energy-dispersive X-ray microscope (SEM–EDX), JEOL JSM 840A, Japan, was used to observe the elemental distribution. The bright points indicate Pd-rich areas. Pore-size distribution parameters were determined by application of the BET method on a Sorptomatic 1900 FISONS Instrument.
2.6 Catalyst test
The reactions were carried out in a 100 ml Parr microreactor model 4593 with a glass liner. The reactor was charged with 0.2 g of supported palladium catalyst, 5 ml of 96% ethanol, 0.25 ml of toluene, and 0.25 ml of cinnamaldehyde. The reactor was flushed several times with pure hydrogen and then the hydrogen pressure and temperature were adjusted to the required level, 40 bar and 75 °C, respectively. The progress of the reaction and product distribution were routinely and quantitatively analyzed by gas chromatography (GC) using a Hewlett-Packard 5990 II gas chromatograph equipped with a thermal conductivity detector. The GC column was HP-50þ (crosslinked 50% Ph Me silicone) 30 m × 0.63 mm × 1.0 μm film thickness. The products were identified by matching their retention times with those of authentic samples. In the recycling tests, after a reaction was complete, the catalyst was filtered off from the reaction mixture, washed three times with 20 ml of hot toluene, dried under vacuum and used for the next reaction.
3 Results and discussion
3.1 Characterization of the polymer supports and immobilized Pd catalysts
To determine the physicochemical properties of the polymer matrices and polymer-supported catalysts, we used a variety of analytical methods. These included IR and X-ray photoelectron spectroscopy (XPS) and the nitrogen BET adsorption method. The chemical structure of the polymers was confirmed by FT–IR. Fig. 2 shows IR spectra of the epoxy resin cured with multifunctional polythiourethane (MPTU) and catalyst DER/MPTU/Pd/scCO2. The characteristic peaks at 1507 cm−1 and 1104 cm−1 confirms the presence of thiourethane linkages. The lack of the absorption peaks of the S–H vibration at 2545 cm−1 and of the isocyanate group at 2272 cm−1 demonstrates the quantitative polyaddition of thiol and isocyanate groups. There is a small peak at 1716 cm−1 and there are no free hydroxyl groups only for this catalyst because in this case the homogeneous catalyst was immobilized on the carrier under supercritical conditions. The shifts and clear changes in the intensity of ether absorption bands at 1011 cm−1, 1024 cm−1, 1085 cm−1 and 1233 cm−1 after catalyst immobilization suggest that ether linkage generated with the opening of each epoxy ring or introduced with a polythiourethane hardener is one of the active center of polymer network (Fig. 3a).
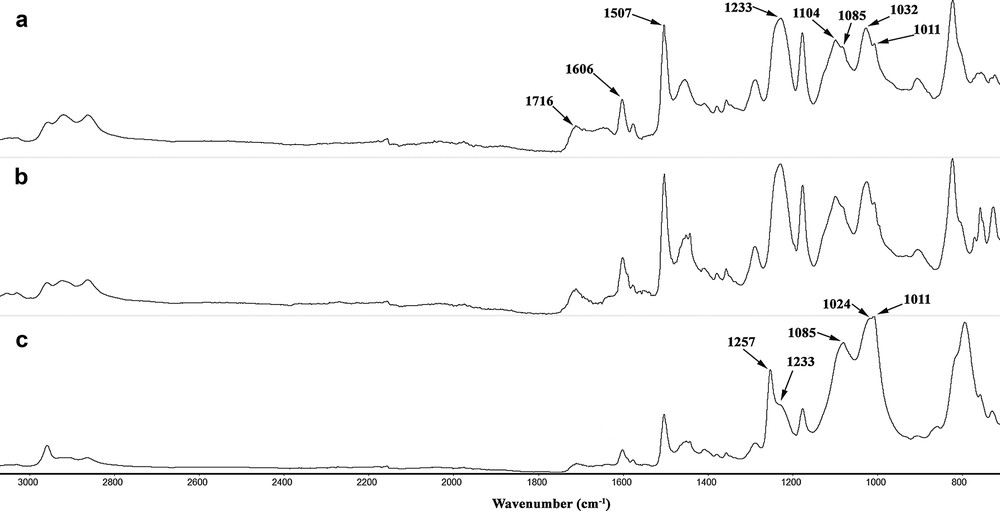
FT–IR spectra of (a) DER/MPTU/Pd/scCO2; (b) DER/MPTU/Pd; c) DER/MPTU.

Possible modes of coordination of the Pd(II) complex by the DER/MPTU support.
The stretching vibration of the C O group of the urethane combined with strong absorption at N–H at 1257 cm−1 and the presence of characteristic urethane bands at 1606 cm−1 attests to the urethane crosslinking process (Fig. 3a). The free hydroxyl groups remaining in the condensed resin provide adequate points for epoxy crosslinking via urethane linkages by the extremely reactive isocyanate groups released through dissociation of thiourethane moieties by the heat of the curing reaction. The shifts in the intensity ratio of these bands indicate an interaction between the nitrogen atom and the transition metal. The change in intensity of bands at 1104 cm−1 and 1507 cm−1 shows that the oxygen and nitrogen atoms of the thiourethane moiety can participate in metal coordination (Fig. 3b). The IR spectra of the polymer-supported palladium catalyst is consistent with the results from XPS measurements. X-ray photoelectron spectroscopy analysis gives a better indication of the chemistry of the surface of the heterogenized catalyst particle, whereas the other analysis methods are more relevant to the composition of the particles as a whole. XPS analyses the outer surface of particulates and enables significant information to be extracted concerning the coordination of a catalytic metal to the polymer support and the mechanism of the catalytic reaction. The binding energy peak of Cl 2p3/2 in the region of 198.8–199.0 eV observed in the XPS spectra (Table 1) indicates the presence of the PdCl2 structure. As a result of the immobilization of PdCl2(PhCN)2, nearly 5% of PdCl bonds and 1.32% of the divalent palladium bonds emerged. Minor fractions of Pd nanoclusters were also observed, which formed due to the lateral overlapping of the d atomic orbitals. They are not stable under normal conditions, but their prolonged life is related to the protection afforded by the polymer matrix. The XPS data summarized in Table 1 show that three possible coordination sites participate in the binding of Pd to the epoxide support. The XPS spectrum of DER/MPTU/Pd/scCO2 and DER/MPTU/Pd catalysts show that palladium appears predominantly in the form of Pd(II). It indicates that this polymeric support chains might stabilize such a catalytically active monovalent metastable species with a binding energy larger than that of the Pd metal. The recovered DER/MPTU/Pd/scCO2 and DER/MPTU/Pd catalysts after a hydrogenation reaction contained also some minor fraction of Pd(0). Since the epoxide matrix cured with the polythiourethane hardener possesses coordination sites of different coordination abilities, it seems very likely that the zero-valent palladium, Pd(0), comes from the reduction of Pd(II) coordinated to the carbonyl oxygen, the weaker of the three coordination sites, whereas new species formed after each reaction run, Pd clusters, come from the reduction of Pd(II) coordinated to the nitrogen atoms.
Binding energy (eV) values inferred from XPS measurements.
Sample | N1s | O1s | Pd 3d5/2 | Cl 2p3/2 | |||
Nimine | Namine | Pdδ+ | Pd(0) | Pd(II) | |||
DER/MPTU | 402.16 | 400.37 | 531.61 | – | – | – | – |
DER/MPTU/Pd/scCO2 before use | 406.18 | 406.68 | 535.21 | 337.45 | – | 339.2 | 198.91 |
DER/MPTU/Pd/scCO2 after use | 406.20 | 406.69 | 535.26 | 336.44 | 336.8 | 339.1 | 198.82 |
DER/MPTU/Pd before use | 405.17 | 403.66 | 534.25 | 336.34 | – | 340.2 | 198.79 |
DER/MPTU/Pd after use | 405.18 | 403.67 | 534.27 | 335.34 | 335.1 | 340.1 | 198.78 |
3.2 Catalytic test
To confirm the high activity of the prepared catalyst and the impact of the structure of the polymer support on stability, the hydrogenation of cinnamaldehyde (Scheme 1) at a pressure of 40 bar and at 70 °C was carried out. The selective hydrogenation of α,β-unsaturated carbonyl compounds into their corresponding α,β-unsaturated alcohols is an important step in the preparation of various fine chemicals such as fragrances for the perfume industry. This reaction can give three products, namely hydrocinnamaldehyde (HCALD), cinnamyl alcohol (CALC), and hydrocinnamyl alcohol (Scheme 1). The mostly desired product is an α, β-unsaturated alcohol that is difficult to produce in the catalytic hydrogenation of α,β-unsaturated aldehydes because the hydrogenation of C C bonds in the presence of noble metals is thermodynamically and kinetically favored over the hydrogenation of the C O bonds. The results (Table 2) show that the morphology, the chemical structure of the polymeric matrix and the conditions of immobilization influence the selectivity of this reaction, which strongly depends on the types of binding groups present in the polymer.
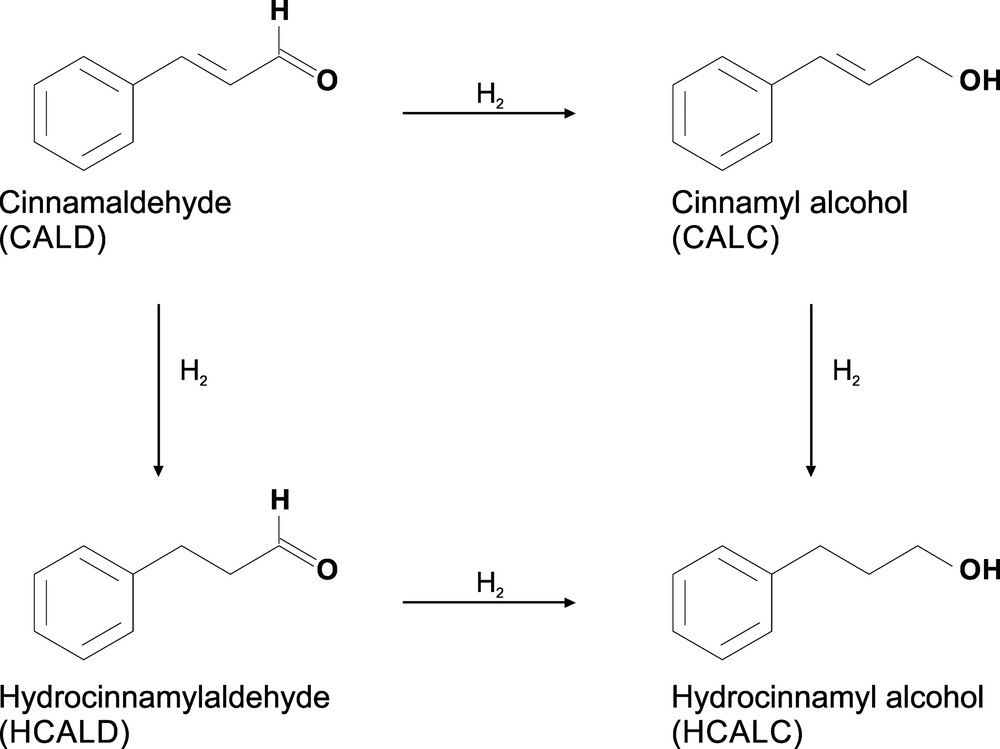
Hydrogenation of cinnamaldehyde.
The hydrogenation of cinnamaldehyde over the DER/MPTU/Pd/scCO2 and DER/MPTU/Pd.
Catalyst | Run | Intensity of 106Pd+ | Conversion [%] | HCALD [%] | HCALC [%] | CALC [%] |
DER/MPTU/Pd/scCO2 | 1 | 7.8 × 10−3 | 100 | 10 | 60 | 30 |
5 | 6.4 × 10−3 | 98 | 3 | 80 | 17 | |
DER/MPTU/Pd | 1 | 4.5 × 10−3 | 97 | 7 | 62 | 31 |
5 | 2.9 × 10−3 | 95 | 3 | 94 | 3 |
The studies show that the catalyst's selectivity depends on the size distribution of the metal immobilized on the carriers. For DER/MPTU/Pd/scCO2, the distribution of the metal complex in the polymer matrix was better, which SEM micrographs confirmed. Fig. 4 shows the elemental distribution for the cases of DER/MPTU/Pd/scCO2 and DER/MPTU/Pd. The micrograph of DER/MPTU/Pd/scCO2 indicates a uniform distribution of the palladium particles. A less regular distribution is observed for the DER/MPTU/Pd catalyst (Fig. 4a,d). Different size distributions of the metal particles, clearly visible on lower magnification SEM–EDX images (Fig. 4c,d), influenced by the morphology of the support, can be an explanation of the unequal catalyst performances of the DER/MPTU/Pd catalyst, for which the immobilization was carried out without the use of supercritical conditions. The results presented in Table 2 show that for catalysts DER/MPTU/Pd/scCO2 and DER/MPTU/Pd, there is a significant difference between the product's distribution between the 1st and 5th runs. This is probably caused by an easier access to the active spots in the open pores of the catalyst. DER/MPTU/Pd/scCO2 catalyst selectivity was slightly lower than for the DER/MPTU/Pd catalyst, which might be caused by the release of the interior of the metal from the swollen matrix during the reaction. The conventionally prepared catalyst showed higher selectivity to HCALC, whereas the DER/MPTU/Pd/scCO2 catalyst had higher selectivity to CALC, which is more desirable.
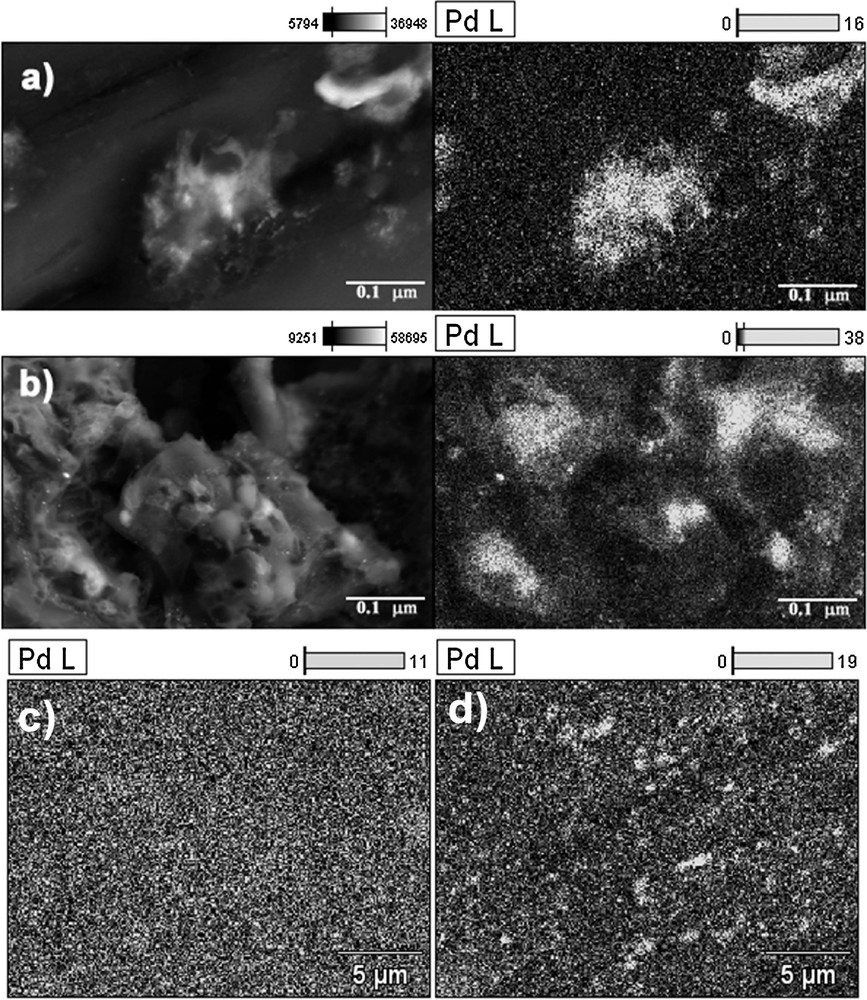
SEM micrographs of the surface of the (a and c) DER/MPTU/Pd/scCO2; (b and d) DER/MPTU/Pd.
Recycling the immobilized palladium catalysts five times demonstrated their high stability, despite the changes that occur in their structure during the catalytic reaction. The selective mercury poisoning was used to exclude the possibility of catalyzing the reaction by unbound palladium metal, which is the result of elution of the complex from the surface of the carrier. This method is commonly used to define the nature of homogeneous or heterogeneous reaction [15–18]. The DER/MPTU/Pd/scCO2 and DER/MPTU/Pd catalysts were exposed to mercury and then subjected to the catalytic tests. The catalytic activity was similar to that against mercury poisoning. For DER/MPTU/Pd/scCO2, we observed a loss of activity after mercury poisoning, which means that the use of scCO2 enables also the introduction of chemically unbounded complexes into the matrix of the support. This test was also done for the homogeneous complex PdCl2(PhCN)2. In this case, the addition of Hg(0) to the homogeneous reaction mixture caused the reaction to stop. Mercury poisoning tests showed that catalysis was not associated with the leached precursor complexes or in situ formed palladium nanoclusters, which would redeposit to the polymer support after the reaction.
3.3 Catalyst stability
The stability of the supported catalyst was tested during repeated catalysis runs. Recycling the immobilized palladium catalysts five times demonstrated their high stability. The time-of-flight secondary-ion mass spectrometry (ToF-SIMS) was applied to investigate the deactivation process and to observe the deterioration of palladium distribution on the catalyst surface after the 1st and the 5th uses of the catalysts. ToF–SIMS method permits the evaluation of the chemical composition of the catalyst surface, of the nature of interactions between the metallic phases and the support as well as the distribution of the metal. For the DER/MPTU/Pd/scCO2 and DER/MPTU/Pd catalysts, a drop of the surface accessible Pd was observed (Table 2) after the 5th run. A small drop of the amount of surface accessible palladium was observed, especially for DER/MPTU/Pd/scCO2; however the drop of the Pd content was at a safe level and did not significantly affect the activity.
3.4 Structure porosity studies
Our studies showed that the morphology of a polymer support can greatly improve and affect the activity and selectivity of the heterogenized catalyst. Steric demands for a catalytic center bound to a support can be different from those of its homogeneous analogues. The structure porosity of the support and the conditions of the immobilization process affect the accessibility of the interior of the swollen resin by increasing diffusional restrictions for the reaction species. The BET nitrogen adsorption method was used to investigate the structure porosity of the investigated materials (Table 3). For all supports, the immobilization of the palladium precursor resulted in a similar loss in surface area and a corresponding decrease in mean pore diameter. The BET data shows that the original structure porosity of the polymer supports has been changed after the attachment of the Pd complex. The catalysts DER/MPTU/Pd/scCO2 and DER/MPTU/Pd shows a pore-size distribution with a lower volume of micropores, and these catalysts had micropores and a greater volume of meso- and macropores. In addition, a significant decrease in specific surface area after the immobilization of metal was observed for the carrier (for DER/MPTU/Pd/scCO2, 155 m2/g before immobilization to 102 m2/g after immobilization). Such a significant decrease in the specific surface area was caused by the metal complex blocked on the DER/MPTU support. We are aware of the poor accuracy of the BET method for low-porosity materials like the highly crosslinked epoxy resin. With regard to the swelling behavior of the resin, especially when the catalytic reaction is performed under solid-liquid heterogeneous conditions, it is important to realize that the dimension of the pores can change enormously. Therefore, in future work, we will apply additional techniques such as inverse steric exclusion chromatography (ISEC) and pycnometry to study the morphology of these supports and catalysts in the swollen state.
Characteristics of polymer support and supported Pd catalysts by the BET methods.
DER/MPTU | DER/MPTU/Pd/scCO2 | DER/MPTU/Pd | |
Surface area (m2 g−1) | 155 | 102 | 75 |
Mean pore diameter (nm) | 2 | 1.9 | 1.9 |
Volume of pores (%) | |||
macro w > 50 nm | 10.9 | 12.3 | 11.1 |
meso 2 < w < 50 nm | 56.7 | 55 | 54 |
micro w < 2 nm | 32.4 | 36.8 | 34.9 |
Pore specific volume (cm3 g−1) | 0.22 | 0.33 | 0.31 |
Porosity (vol vol−1) | 0.25 | 0.36 | 0.32 |
4 Conclusion
The results of this study have demonstrated that the prepared polymer support meets the requirements for organic supports and can be used for the preparation of palladium catalysts. Additionally, the use of supercritical conditions cause a better distribution of the homogeneous catalyst in a matrix of polymeric support and influence the activity of the catalyst prepared in scCO2 The immobilization in scCO2 conditions is a simple method and allows a high concentration of metal in the carrier matrix as compared to the traditional method of immobilization. The immobilization in scCO2 can be used even for carriers with low sorption capacity, because metal can get into the deep layers of the matrix, which is not available with standard immobilization. Thus, it could find a possible use as a convenient procedure for the preparation of industrial catalysts. The use of polythiourethane as a curing agent enables one to obtain supports that do not need any further functionalization. The investigated catalyst showed good activity and selectivity during prolonged use in the cinnamaldehyde hydrogenation reaction.
Acknowledgement
This work was supported by the National Science Centre (Poland)—grant No. 0255/B/H03/2011/40.