1. Introduction
Delivering small molecule or peptide drugs, biologics, or any viable cells in a safe, painless, targeted, efficient, and cost-effective way remains a central concern for all researchers and developers of future medicines. However effective an active compound may be, if it is not properly administered, and/or delivered to the right site and at the right time, with the right dose, all its effectiveness may be lost. This explains why there is a long way to go between tests carried out ex vivo on cells, in vivo with laboratory animals and finally in human beings with their own heterogeneity and complexity. Another challenge that has to be met is to resist stomach acid and pass biological barriers (skin, pericardium, blood–brain barrier [BBB], mucous membranes, tears, earwax, mucus) that are heterogeneous across patients and can be impaired in diseases. It should be noted that for certain applications, the passage of natural barriers, for example to the brain, is not at all desirable.
In recent years the quest to refine systems allowing the targeted delivery of drugs has exploded and led to extraordinary developments, especially by using “intelligent” nanoparticles (NPs) for applications in nanomedicine, therapy, and diagnostics [1, 2, 3, 4, 5, 6, 7, 8, 9]. NPs present a large spectrum of advantageous properties for biological and medical applications. They can be designed to have specific surface properties that allow them to selectively target abnormal cells, for example, tumor cells [10], while ignoring healthy ones, thereby reducing deleterious effects of otherwise efficient drugs. NPs can be engineered to liberate their cargo in a controlled manner, slowly or rapidly, enabling the drug to be released at the right time, at the intended site, for example, an inflammatory site, a tumor, or an infected tissue. NPs also have decisive applications for diagnostic purposes, as contrast agents (CAs) or tracers in medical imaging or, for example, as detection probes of specific biomolecules in biological samples [3, 6]. Finally, they can be internalized in cells to ensure hyperthermia-mediated treatment of cancer [11].
If it is largely recognized that in general NPs allow to improve drug targeting, reduce side effects, and diminish potential toxicity concerns of certain drugs, we cannot ignore that ethical considerations related to safety access and affordability surround the use of NP-based drug delivery systems [12, 13, 14]. We need to take account of the fear felt by some patients, their families, and certain opinion groups. In this review, we present the progress in NP designs applied in both general and precision therapeutic applications in tracking analyses and theranostics. We highlight the progresses that have been made in recent years and some limitations that represent the future challenges to pass in the next years. This field of research and development is eminently cross-disciplinary. Constant progresses in all areas (chemistry, material science, biophysics, pharmacology, cell biology, cellular immunology, biomedical sciences) must merge to innovate and produce increasingly effective and safe nanotools.
2. Nanoparticles: Which one for diagnostics, tracking analysis, and for therapy?
The ability to finely shrink materials to nanoscale dimensions from 1 to 100 nm has led to the production of NPs that exhibit unique structural, chemical, and biological properties, paving the way for a new field of research [15]. Understanding the influence of NP properties on clinical translation issues such as pharmacokinetics, pharmacodynamics, bioaccumulation, excretion mode, and toxicology is of utmost importance [16]. These parameters classically assembled under the general term “critical nanoscale design parameters” include their size, shape, surface functions, rigidity or flexibility, architecture (their design), and elemental composition influencing their biodegradation, biodistribution as well as their immune recognition.
Over the past two decades, the development of functional NPs has progressed exponentially into a broad range of applications in biology, technology, and medicine. As mentioned above, successful applications especially include targeted therapy, and their use as molecular probes, aiding in cell tracking and imaging diagnostics [17] (Figure 1).
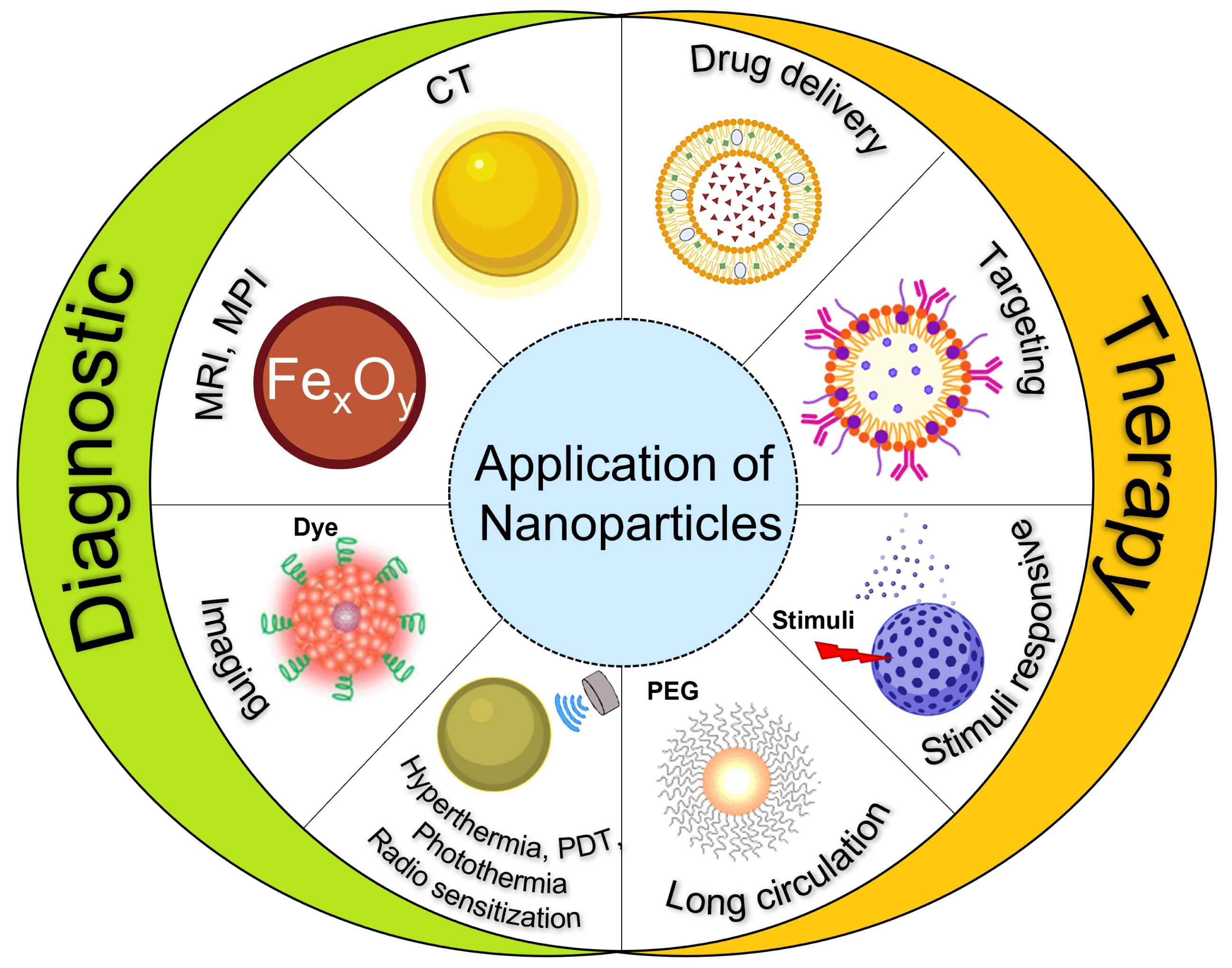
Application of nanoparticles. Photodynamic therapy (PDT), magnetic resonance imaging (MRI), magnetic particle imaging (MPI), and computed tomography (CT).
Labeling cells, such as stem cells, with NPs enables tracking their path in the body and identifying cell interactions [18]. Furthermore, NPs can be used as CAs or tracers to improve the imaging of internal organs, for instance, aiding in the early detection of tumors [19] or specific area of gut inflammation [20]. The application of NPs in cell targeting exploits several of their unique features, namely their small size, the high surface-to-volume ratio, and their chemical functionalities [21]. Numerous articles have been published describing these aspects, guiding us toward the choice of the right matter for the right purpose [22]. According to a recent survey [23], the most commonly used NPs for cell tracking and diagnostic purposes are inorganic NPs, accounting for 79% of the total work relating the remarkable properties of NPs. In this class of material, the most common are iron oxide and gold NPs (Figure 2).
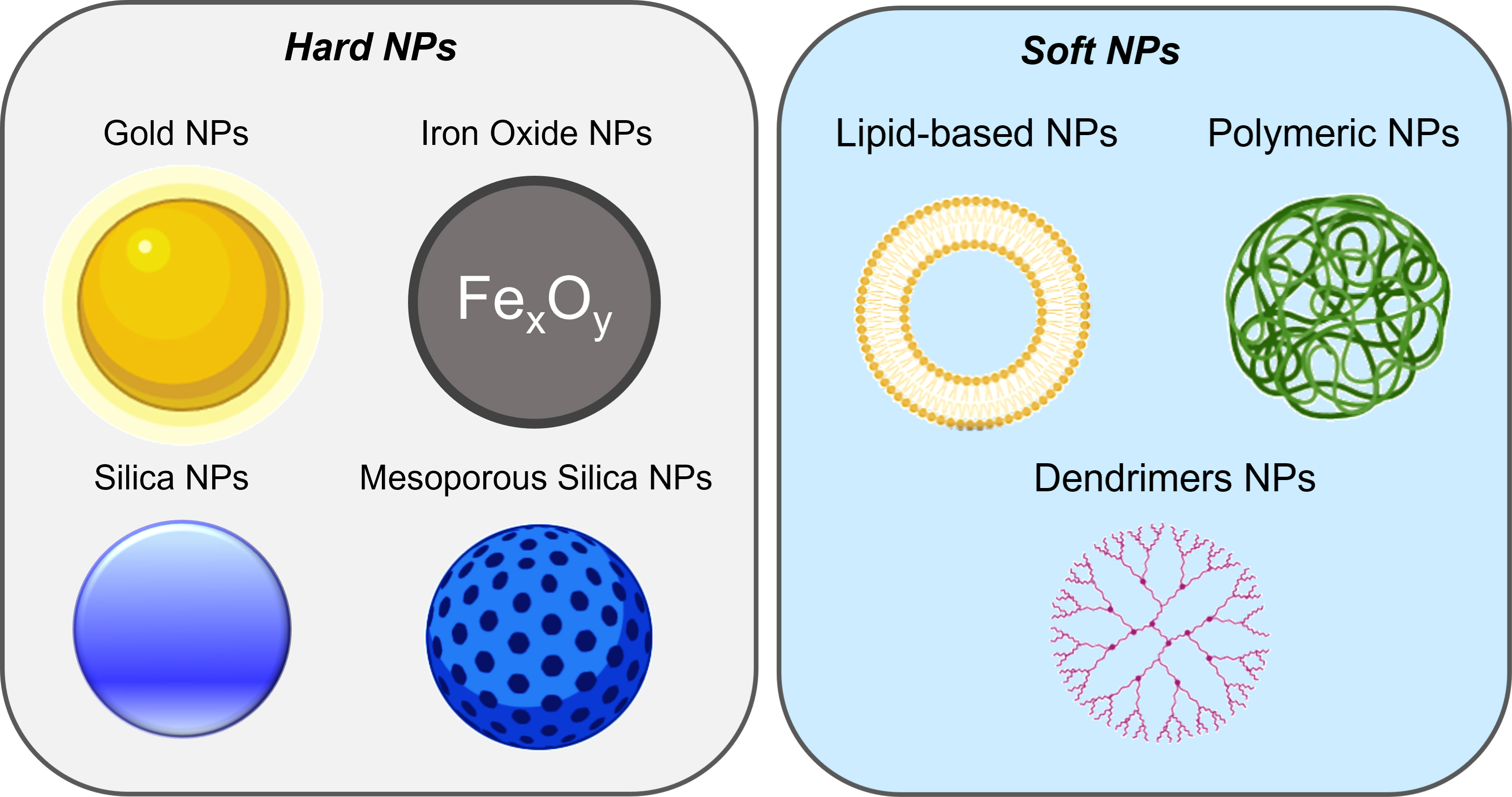
Schematic illustration of different classes of NPs. Based on the material composition, NPs are classified into hard and soft NPs. Hard NPs include gold NPs, iron oxide NPs, and silica and mesoporous silica NPs. Soft NPs include lipid-based NPs, polymeric NPs, and dendrimers.
2.1. Iron oxide NPs
The first example of potential inorganic NP candidates are iron oxide NPs (IONPs), which are mainly composed of magnetite (Fe3O4), maghemite (γ-Fe2O3), and mixed ferrites [24]. IONPs combine magnetic properties and low toxicity, providing the advantage of delivering high-resolution images of human soft tissue via magnetic resonance imaging (MRI) in a safe way [25, 26]. Therefore, IONPs have already been exploited commercially as T2 (see footnote1 ) CAs for MRI [27], and are of particular interest as biodegradable nanoobjects compared to other CA families [28]. They can also be used as magnetic particle imaging (MPI) tracers [29] or as a magnetic particle hyperthermia tool to treat cancer by heating solid tumors to 41–47 °C for a defined time to achieve a prescribed thermal dose [30]. To date, a type of amino silane-coated superparamagnetic IONP (SPIONs), called Nanotherm was the only formulation approved in magnetic hyperthermia for the treatment of primary or recurrent glioblastoma multiform and is now in clinical trials to treat prostate cancer [31].
Combining imaging and therapeutic properties, IONPs are thus prime candidates for a theranostic approach to nanomedicine [32]. Ultrasmall SPIONs (USPIOs) (<5 nm) showed reduced longitudinal and transverse relaxation times, offering a better T1 contrast (brighter images; see footnote 1) where they accumulate, comparable to the common gadolinium-based CAs [24, 33]. Moreover, negatively charged USPIOs also showed a tropism to activated macrophages in highly inflamed areas avoiding off-target accumulation, suggesting their safer use in targeting imaging of inflammatory disease states [33]. Functionalization of IONPs has resulted in efficient cellular uptake and reduced toxicity [34]; indeed, to improve their internalization in cells lacking in phagocytic capacity as mesenchymal stem cells, different positive coatings were used such as poly-L-lysine, chitosan, 2-aminoethyl-trimethyl ammonium, amphiphile lipopeptide, and poly(lactic-co-glycolic) acid (PLGA) [35]. In terms of active targeting to boost NP uptake in cancer cells, IONPs have been functionalized with moieties such as transferrin and its analogues, antibodies (e.g., targeting epidermal growth factor receptor [EGFR], endothelial growth factor, herceptin, or CD44, involved in cell–cell interactions, cell adhesion and migration), aptamers (single-stranded nucleic acid ligands or peptides that bind to target molecules with high affinity and specificity), hyaluronic acid, folates, glucose, and peptides including chlorotoxin, Arg-Gly-Asp peptide (RGD), and Glu-Pro-Pro-Thr peptide [36].
2.2. Gold NPs
Among metals, gold is emerging as a promising material for imaging, diagnosis, and cell tracking. Gold NPs are biologically inert, biocompatible, easily tailored with functional groups, and their size and shape are highly tunable [37, 38]. In vivo studies confirmed that gold NPs can passively accumulate in tumor or inflamed sites through enhanced permeability and retention effect [39].
With the advancement of new therapies as cell-based therapy, there is an increasingly urgent need for methods capable of monitoring the injected cells to determine their fate and functions. Computed tomography (CT) is a non-invasive imaging technique widely used in clinics that provides three-dimensional anatomical information of specific organs and improves diagnoses and treatment efficacy. One of the limits of CT is its lower sensitivity toward CAs [40, 41]. As a consequence, the large doses of iodine-based CAs needed can cause allergy reactions and kidney toxicity in patients [42]. Some of these limitations have been overcome using newly emerging gold NPs-based CAs [43, 44]. Various gold NPs have been investigated for CT imaging applications in tumors, thrombi, kidneys, and bones. Due to their high flexibility in coating and targeting, ligand-decorated gold NPs exhibit enhanced tissue-specific molecular reactivity compared to iodine or other nanomaterials. Notable examples include gold NPs conjugated to anti-integrin RGD tripeptides, anti-EGFR-2 as well as peptides such as the prostate-specific membrane antigen, RNA aptamers, and folic acid, all of which demonstrate improved accumulation in tumor cells [39]. Successful examples of immune cell tracking (e.g., dendritic cells and T-cells) include the use of gold NPs coated with glucose molecules and conjugated to anti-Programmed Death-Ligand 1 (PD-L1) antibodies, thiolated adenine-rich oligonucleotides, and poly(amidoamine) dendrimers [45, 46, 47, 48]. Similar to IONPs, it is important to note that other physico-chemical properties such as size and shape significantly influence cellular uptake and their biodistribution. Gold NPs smaller than 6 nm are rapidly excreted by the kidneys [49] while ∼13 nm gold NPs show slower tissue penetration but increased accumulation over time, compared to larger, ∼50 nm gold NPs. Moreover, gold NPs exhibit shape-dependent bioaccumulation; for example, star-shaped gold NPs were shown to display similar kinetics to spherical gold NPs in the liver and spleen but a distinct tropism to the lungs [50].
NP systems represent also a successful tool for drug delivery. Although some hard NPs, such as mesoporous silica, are used for this purpose [51], most of the strategies are based on soft materials such as lipid NPs and polymeric NPs [52, 53]. These NPs present an empty cavity where the therapeutic molecule is hosted and protected from degradation.
2.3. Lipid NPs
Lipid nanoparticles (LNPs; Figure 2) have gained broad use in medical research. Since Doxil®, the first liposome doxorubicin nanodrug, was approved for the treatment of different types of cancers, several nanoformulations (DaunoXome, AmBisome, Myocet, and DepoCyt) have been released on the market, especially in the context of cancer immunotherapy [52]. In addition to their application in oncology, LNPs have recently been approved for the delivery of mRNA and small interfering RNA (siRNA) in coronavirus disease (COVID)-19 vaccines and amyloidosis treatment, respectively [54]. Recently, Ferraresso et al. tested clinically approved LNP formulations, in a swine model, carrying mRNA to demonstrate the expression of exogenous mRNA and protein synthesis in non-reticulo-endothelial system organs without eliciting over toxicity [55].
LNPs are based on lipid molecules such as natural or synthetic phospholipids, which form the backbone of spherical vesicles, cationic or ionizable lipids, which allow the attachment of negatively charged molecules such as nucleic acid and PEGylated lipids that increase the “stealth” property, thus increasing stability and circulation time in biological fluids. Due to their nature and biomimetic architecture, LNPs are considered highly biocompatible and the least toxic NPs [56]. LNPs are highly versatile drug delivery systems that can encapsulate different types of therapeutic agents. This exceptional property is linked to their unique structure: hydrophilic drugs in their core, hydrophobic drugs within the phospholipid bilayers, and amphiphilic drugs in both regions [57]. As for the other NPs, their size, charge, and capacity to accept surface modifications can influence their biodistribution in a decisive manner. Extensive literature has documented how physico-chemical properties can influence cellular uptake and tropism to areas of inflammation. For example, small NPs (<100 nm) have high rates of cell internalization and extended circulation time while in contrast, larger NPs that show a lower cell uptake can offer higher drug loading capacity. A high surface charge (negative or positive) has been demonstrated to reduce particle aggregation favoring the monodisperse and stable system [38, 58]. Furthermore, surface antibody conjugation [59], cell-specific moieties such as E-selectin/CD62E [60], a cell adhesion molecule expressed on endothelial cells, or aptamers [61] represent a good strategy to improve the targeting. In this sense, Kim et al. used dual-aptamer-conjugated liposomes to target mucin 1 and CD44 antigen (a cell-surface glycoprotein involved in cell–cell interactions, cell adhesion and migration, especially of tumor cells) present on both breast cancer and cancer stem cells, respectively [62]. The functionalization and the shield offered by LNPs promote both the selective targeting and drug integrity. This strategy was used to encapsulate immunomodulatory drugs such as Janus kinases and nuclear factor-kappa B inhibitors, viral and self-antigens, and microRNA (miRNA) and siRNA [38, 63]. Similarly, Paoletti et al. found an efficient way to deliver small RNA to monocytes and macrophages reducing joint inflammation in the mouse model of rheumatoid arthritis. AntagomiR-155-5p encapsulated in PEGylated LNPs presented the advantage to selectively target and correct the defect of polarization of monocytes into anti-inflammatory macrophages [64]. Controlling the drug release is another challenge that has been achieved with LNPs by engineering their backbone to be sensitive to diverse stimuli, such as redox environment, presence of specific enzyme, and change in pH or hypoxia (i.e., when levels of oxygen are low in body tissues) [65].
2.4. Polymeric NPs
Due to their remarkable versatility, polymers, both natural and synthetic, have been extensively utilized in the development of nanometric colloidal systems (Figure 2). These systems offer numerous benefits, including biodegradability, biocompatibility, and low toxicity [4]. Encapsulating therapeutic molecules within polymeric NPs has been demonstrated to enhance drug bioavailability, extend the release profile, safeguard the cargo from enzymatic degradation, and minimize cytotoxicity [66].
The use of biodegradable polymers or labile cross-linkers represents an interesting approach for making degradable polymeric carriers. For example, extensive studies have been conducted using natural polymers such as chitosan, alginate, gelatin, and albumin [53]. A groundbreaking development in the formulation of paclitaxel (PTX) was marked by the market approval of Abraxane, an albumin NP formulation loaded with PTX. Clinical data clearly demonstrated the superiority of this NP-based approach, particularly in reducing PTX toxicity, which allowed for a higher maximum tolerated dose in cancer patients [67]. Several other polymers with biodegradable properties have been largely described in the literature, including PLGA, poly(ε-caprolactone), and many others [68, 69]. Such polymers were applied to promote the gradual release of cargo (e.g., immunosuppressant agents), fostering the reduction of inflammatory signals in autoimmune and acute inflammatory diseases [70].
Polymeric NPs can encapsulate different types of cargo including hydrophilic and hydrophobic drugs, proteins, and nucleic acids. As described for other NPs, surface-bound targeting ligands can enhance the delivery of drugs to specific sites and bind pro-inflammatory cells. For instance, PLGA NPs were designed to attach to tumor necrosis factor receptor 1 on macrophages and intercellular adhesion molecule 1 on endothelial cells at the site of disease [70]. Zhang et al. described experimental results showing that galactosylated chitosan NPs achieved significantly higher uptake by intestinal activated macrophages in a mouse model of inflammatory bowel disease [71].
Dendrimers are an important group of nanostructured polymeric carriers for the development of nanomedicine to treat various diseases [72]. Because of structural diversity and adaptability, they have been used to deliver drugs and genes in many ways. For instance, dendrimers with a hydrophobic core and a hydrophilic periphery may behave like unimolecular micelles, and they have been utilized to solubilize hydrophobic drugs by entrapping them in the intramolecular cavity [73]. Cationic dendrimers have been extensively applied as non-viral gene carriers [74]. Dendrimer surface groups can be conjugated with drugs and other functional moieties [75].
2.5. Mesoporous silica NPs
Among hard NPs that show promises for drug delivery, mesoporous silica NPs (Figure 2) deserve particular attention. They can be synthesized to give rise to NPs of different sizes, shapes, and levels of porosity. Their porous structure allows for effective drug loading and provide drug protection, while the outer surface can be further functionalized with polymer, dyes, and targeting molecules [76]. Notably, silica was defined as “Generally Recognized as Safe” by the Food and Drug Administration (FDA), and several clinical trials are currently underway utilizing silica-based NPs [51]. An example of such a class of NPs is ultrasmall silica NPs (6–10 nm in size) called Cornell dots, which are under evaluation in clinical trials (ClinicalTrials.gov identifier/NCT number NCT03465618, NCT01266096, and NCT02106598). Their small size allows clearance by the kidney. They demonstrated good uptake by sentinel lymph nodes in patients with head or neck melanoma to allow visualization during biopsy. In another study, the functionalization with tumor-homing arginyl-glycyl-aspartic acid-tyrosine (RGDY) 4-mer peptide and fluorescent dye Cy5.5 showed good uptake by tumor cells and an improvement by 20 to 30 times for the diagnosis and staging of melanoma and malignant brain cancer [51].
In parallel to mesoporous silica NPs, extensive investigations have been focused on mesoporous organosilica NPs (Figure 2). The presence of organic moieties (disulfide, amide, oxamide, imine groups) in inorganic silica lends itself to the characteristic of breakability under precise environmental conditions controlling the release of the cargo while retaining the features of silica NPs [78, 79, 80]. In this context, we developed a valuable “Trojan horse” system based on breakable silica-cage-like NPs (OrganoSilica nanoCages [OSCs]) able to avoid macrophage uptake and specifically targeting liver sinusoidal endothelial cells (LSECs). This nanotool holds the possibility for LSEC modulation, impacting cellular and immunological processes such as tumor cell extravasation, hepatic immunity, and tolerogenic state of immune cells in autoimmune diseases [81] (Figure 3).
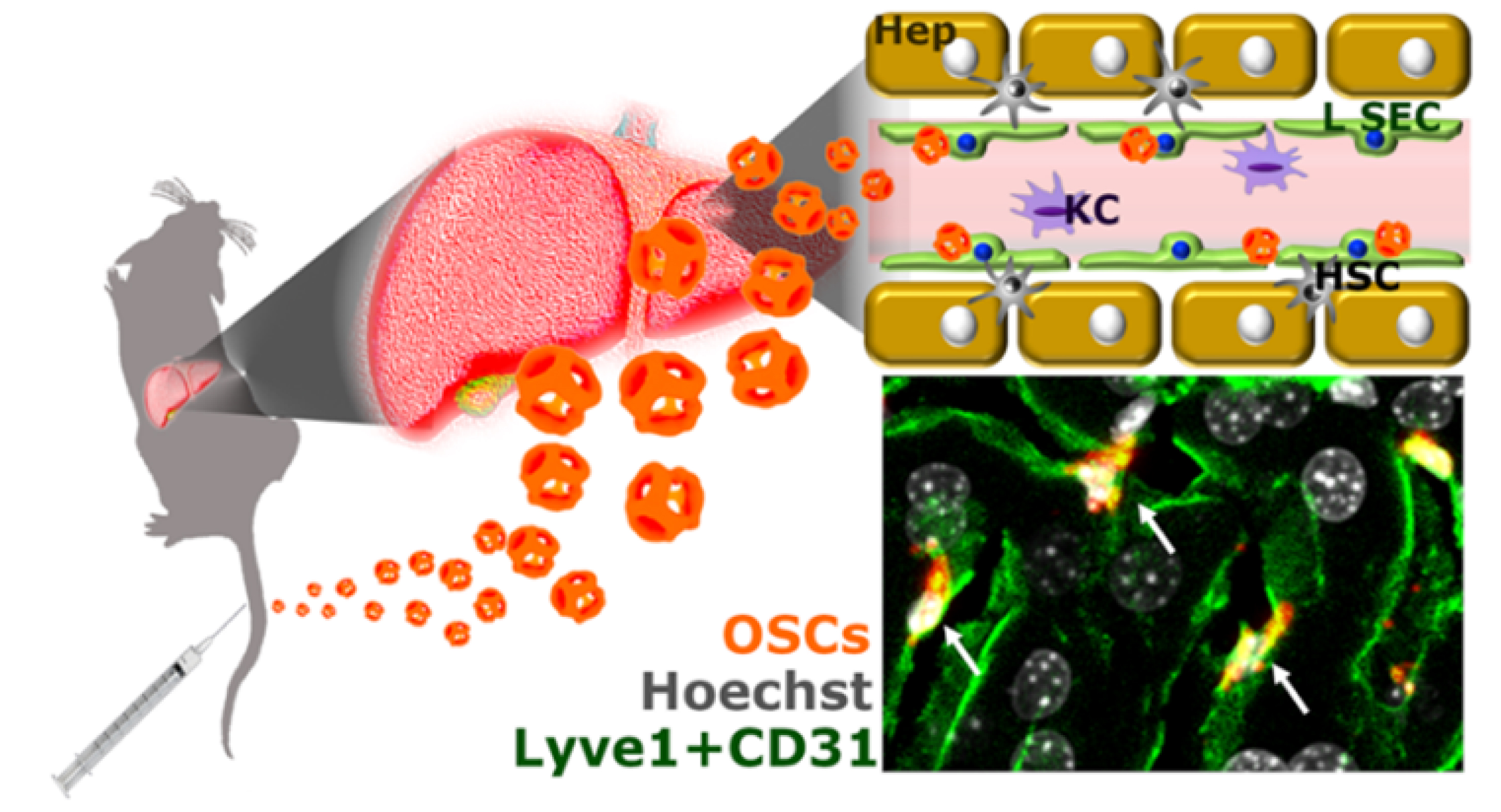
Schematic illustration of OSCs administered in tumor-bearing mice and their ability to escape from macrophage uptake and exclusively target LSEC. Images depicted in green are lymphatic endothelial cells, in red are NPs, and in gray are nuclei (adapted from [77]).
Altogether these data illustrate that according to the final purpose of the project (therapy, diagnostics, tracking, or targeting), the type of in vitro or in vivo models that are exploited, the localized or systemic receptors that are targeted, and the dynamics of the process that is required, a variety of nanomaterials are available that can give excellent results if selected properly. The choice of tools designed to follow the NPs, once they are administered into experimental animals, is also multiple (Figure 1). However, some technical limitations remain to solve for certain applications such as transdermal drug delivery or for magnetothermal brain stimulation to mention just two examples, leading to intensify research further to fill these gaps.
3. Magnetic nanoparticles for in vivo cell and extracellular vesicle therapy tracking
Advanced therapy medicinal products (ATMPs) will be tomorrow what transfusion is today: personalized medicine, based on expert knowledge of cells or extracellular vesicles (EVs) to reverse disease. For example, regenerative medicine, based on the transplantation of cells produced in laboratories, is no longer the distant dream of a few researchers, but a clinical reality that is being applied in numerous therapeutic trials (https://clinicaltrials.gov). The time has therefore come to anticipate the deployment of this new medicine to all patients, whether affected by neurodegenerative pathologies, autoimmune diseases, or cancer.
There is growing interest in cells and EV labeling using exogenous markers such as NPs to identify and monitor in real time in vivo migration of transplanted therapies. Indeed, one of the issues encountered in the field of ATMPs is the lack of information on the biodistribution of cells and/or EVs once injected into the patient. Therefore, non-invasive imaging methods such as MRI, for example, should enable to better understand the functions of implanted cells [82], assess the efficacy of cell therapies [83], and determine which therapeutic protocols give the highest probability of regeneration or healing. Moreover, ensuring disruptive monitoring of ATMPs in vivo through MPI would not only enable quantification [84] for possible dose reduction but also mitigate the risk of cell therapy escape, with the possibility of destroying injected cells in the event of uncontrolled multiplication via the use of theranostic NPs and focalized magnetic hyperthermia [85].
Various techniques can be used to track ATMPs. In vitro, the most widespread approaches are the use of bromodeoxyuridine or green fluorescent protein [86] for the tracking of implanted cells on histological examinations. In vivo, two types of tracking are possible, either direct imaging or indirect tracking. In the case of indirect monitoring, this involves using a reporter gene (an exogenous coding region joined to a regulatory sequence of another gene of interest) [87]. In the case of a direct follow-up, the tag is internalized before the cells are injected. Several imaging modalities are then possible, namely non-invasive MRI [88] via the internalization of SPIONs (1H-MRI) or perfluorocarbon derivatives (19F-MRI) [89]. The latter option enables in vivo quantification and thus to join MPI or nuclear imaging, such as positron emission tomography [90], and single photon emission computed tomography [91]. Immune cells and bone marrow (BM)-derived stem cells were initially labeled with an 111In-oxine radiotracer to determine their biodistribution in patients using γ-camera imaging [92]. For the first time, it was thus possible to visualize cells in the whole body without the need of biopsies. However, radiotracers can exhibit significant cytotoxicity and lack an infinite half-life. Furthermore, nuclear imaging techniques do not provide anatomical information and high spatial soft tissue contrast. This led to the development of alternative non-invasive in vivo cell tracking approaches with MRI historically being one of the primary clinical imaging modalities [66], followed by MPI as a more recent preclinical cell tracking technique [93].
Focusing on magnetic tracers, if properly used, SPIONs do not alter viability, function, proliferation, or differentiation of cells [77]. However, an issue is possible as the tag may be transferred to local, bystander cells such as tissue macrophages [94], especially in the areas of inflammation, thus leading to confounding the microscopy or imaging interpretations. This is of particular importance in direct implantation of cells into target tissues, which can result in 50% to 80% cells undergoing cell death [95]. However, a few studies showed that transfer of iron to activated macrophages accounted for less than 10% of the total iron in labeled cells [96].
3.1. Tracking cells with SPIONs
In 2018, H. Daldrup-Link and co-workers published a proof-of-concept study in patients on the tracking of autologous BM-derived cell transplants in femoral osteonecrosis with MRI [97]. This kind of clinical imaging test is important as it may help to recognize inter-individual differences in the delivered quantity and location of transplanted cells, and correlate results with tissue repair outcomes. It could become a powerful new tool to monitor the delivery and engraftment of BM-derived therapeutic cells non-invasively in cancer patients with the ability to directly impact patient management. The same year, another group used a clinically applicable approach combining the FDA-approved drugs heparin, protamine, and ferumoxytol (a USPIO) to form nanocomplexes for magnetic Natural Killer (NK) cell labeling so that NK cell biodistribution could be visualized in vivo with MRI following transcatheter intrahepatic arterial (IHA) local delivery [98]. The purpose of these studies was to test several hypotheses in a rat model of hepatocellular carcinoma, and to determine if (1) clinically applicable labeled NK cells can be tracked with MRI, (2) transcatheter IHA NK infusion can improve NK cell homing efficacy to targeted tumors, and (3) serial MRI monitoring of NK cell migration to targeted tumors can serve as an early biomarker for prediction of longitudinal response.
More recently, H. Daldrup-Link and colleagues successfully labeled Chimeric Antigen Receptor (CAR) T-cells with ferumoxytol, thereby paving the way for monitoring CAR T-cells in solid tumors [99]. This work demonstrated the feasibility of approaches using mechanoporation via a microfluidic chamber to label CAR T-cells with IONPs reaching a substantial labeling efficiency. It was then possible to detect the transferred T-cells using several imaging modalities, for example, MRI, photoacoustic imaging, and MPI. Such imaging approaches, which allow monitoring the localization of the ATMP in a dose-dependent manner, may contribute to elucidating the factors that give rise to responders and non-responders of T-cell therapy against solid tumors.
3.2. Extracellular vesicle tracking
Human stem-cell-derived EVs are currently being investigated for cell-free therapy in regenerative medicine applications, but the lack of non-invasive imaging methods to track EV homing and uptake in injured tissues has limited the optimization of the approach. In 2021, G. Liu and co-workers, at Johns Hopkins University, developed an electroporation labeling strategy to prepare magnetic EVs allowing MRI tracking of systemically injected therapeutic EVs. Relying on the use of “sticky” SPIONs coated with a poly-histidine tag, this new labeling strategy allowed to efficiently separate magnetic EVs from unencapsulated SPIONs particles. Using this method, MRI tracking of the homing of systemically administered pluripotent stem cell (iPSC)-derived magneto-EVs in different animal models of kidney injury and myocardial ischemia was possible and showed that iPSC-derived EVs preferentially accumulate in injury sites and confer substantial protection [100].
In summary, perspectives in the monitoring of ATMPs lie in combined approaches such as MRI/MPI or MRI/19F-MRI/MPI for an efficient quantification without the use of radiation [101], which has proven efficient for tracking adoptive T-cell immunotherapy [102] or for monitoring the delivery of mesenchymal stem cells and the ensuing inflammation [103]. Indeed, quantitatively monitoring mesenchymal stem cell engraftment and ensuing inflammation over time would be invaluable for evaluating the course of therapy.
4. Nanoparticles and immunotherapy
Immunotherapy treatments include monoclonal antibodies, checkpoint inhibitors, cytokines, vaccines, and CAR T-cell therapy. Often used in the context of cancer, immunotherapy approaches are also used in the case of infection and immune diseases, including autoimmunity. In cancer, adoptive cellular therapy increases the number or the effectiveness of immune cells, usually T-cell engagers, which improves the power of the immune response against tumor cells [104]. Immune checkpoint inhibitors, for example, ipilimumab (Yervoy®) that blocks cytotoxic T-lymphocyte antigen 4 (CTLA-4), pembrolizumab (Keytruda®) and nivolumab (Opdivo®) that target PD-1, and atezolizumab (Tecentriq®) that blocks PD-L1, prevent checkpoint proteins from binding with their partner proteins. This precludes the “off” signal from being sent, allowing T-cells to kill cancer cells. Cytokine-based immunotherapy drugs target cytokine activity to the tumor microenvironment or to the desired effector immune cells [105, 106].
Potentiating the effects of these biological and chemical tools is central not only for increasing their local efficacy but also, as introduced above in this review, for protecting the active compounds from partial or full degradation on the way to their destination and to send them to the right target. The challenge is huge because most tumors with poor prognoses are not always locatable or accessible. Conversely, in the case of immune system disorders, especially in systemic autoimmunity, abnormal infiltrating immune cells are spread throughout various organs. The percentage of T- or B-cells with precise antigenic specificity is however extremely low. In these examples in cancer and autoimmunity, therefore, a local therapy may be ineffective unless a key organ or tissue is identified or a cell subgroup is shown to be central to the regulation of other cell subtypes (as it is the case in the abscopal effect [107, 108]).
It is beyond the goal of this short review to extensively describe the many successful examples and also the failed preclinical studies that have exploited NPs in immunotherapy protocols. Comprehensive reviews have been recently published on this topic [6, 8, 104, 109], and today a growing number of NPs have been approved by the FDA for applications in nanomedicine [4]. Below, we selected a few examples in which remarkable results were obtained these last few years.
Schudel et al. employed a two-stage delivery strategy to enhance the uptake of NPs by cells within lymph nodes, which are typically difficult to target with conventional drugs. In this approach, CpG oligonucleotides were conjugated to 30 nm poly (propylene sulfide) NPs using a degradable linker. This strategy resulted in increased uptake by dendritic cells, T-cells, and cancer cells within the lymph nodes. Additionally, by modulating the fragmentation half-lives of the linker, the researchers were able to control the timing of the antigen release, improving the precision and effectiveness of the delivery [110]. T-cells are another key cell type to target. Polymer NPs coated with DC membrane and conjugated with anti-CD3 antibodies demonstrated efficient accumulation in draining lymph nodes. These NPs effectively activated and expanded CD8+ T-cells and, when combined with anti-PD1 therapy, successfully elicited anti-tumor immunity [111].
Recently, Tamming et al. formulated an LNP-based DNA vaccine encoding the Delta spike protein of severe acute respiratory syndrome coronavirus 2 (SARS-CoV-2). The administration of this nanosystem enhanced the antibody response, providing strong protection against subsequent infections. The vaccine reduced viral replication, prevented lung tissue damage, and downregulated inflammatory pathways, highlighting the potential of this nanosystem for broad protection against various variants [112].
In another field, LNPs were used recently in a research context on pancreatic ductal adenocarcinoma (PDAC) to encapsulate and deliver Stimulator of Interferon Gene (STING) and Toll-Like Receptor (TLR)4 agonists [113]. The authors showed that combining localized immune agonist delivery with systemic tumor-targeted therapy could coordinate innate and adaptive immune responses with durable anti-tumor efficacy in PDAC. These very promising results obtained using syngeneic transplant and autochthonous PDAC mouse models deserve much attention for future applications.
NPs were also applied to correct some lysosome defects that frequently occur in inflammatory, autoimmune, neurodegenerative, and metabolic diseases, as well as in cancer [114]. In the case of Parkinson’s disease (PD), acidifying NPs have been shown to slow neuron death in a mouse model of the disease [115]. These NPs corrected the pH of lysosomes within dopaminergic neurons, which are the first to be affected by PD. In the case of cancer, different strategies utilizing diverse types of NPs have also been described to treat tumors by destroying lysosomes or realizing lysosomal escape [116]. LAMP1-SPIONs (LAMP1 plays important roles in lysosome biogenesis, lysosomal pH regulation, autophagy, and cholesterol homeostasis) induced exosmosis of lysosome contents, meaning they move from the inside of the vesicle, across the semipermeable lysosomal membrane, to the external medium, for example, the cytoplasm. This leads to a decrease in intracellular pH, which would eventually cause apoptosis [117]. Other applications of NPs toward lysosomes are detailed by Tian et al. [116]. These lysosome-oriented strategies are extremely promising since in recent years, lysosomal changes and dysfunction have been correlated with the development of numerous diseases, which has led researchers and developers to propose that targeting lysosomes in human diseases may represent a novel therapeutic way of intervention [114, 118].
One of the most complicated challenges in the field of NPs is their passage through the BBB. Some studies based on phytochemicals have shown that NPs not only improve the pharmacological effect of these substances but also enable targeting the brain and crossing of the BBB. Various ligands were added to NPs to improve BBB transportation, and, for example, a potential to protect against key features of PD was observed, including α-synuclein aggregation, mitochondrial dysfunction, and dopaminergic neuronal death [119].
Many more examples of successful results may illustrate the importance of NP-based strategies in immunotherapy. An important message that emerges from these studies is that they have all required a meticulous analysis of all the parameters, on a case-by-case basis. No global recipe is applicable, and only experimentation has made it possible to identify the best conditions (type of NPs, administration kinetics, route and frequency of injections, timetable, etc.). Slight adjustments in ligand graft density or encapsulation, the length of the ligand–membrane linker, its nature, and all kinds of such parameters can significantly influence interaction with the ligand receptor and consequently the effectiveness of the loaded NP.
An important aspect is also to avoid any problem of immunogenicity of the NP construct [38]. It is effectively of prime importance to develop NPs that are “furtive” with regard to the immune system of the recipient. Anti-drug antibodies (ADAs) that can appear with time in treated individuals may inactivate the drug (e.g., a protein, a therapeutic antibody, a peptide, a small molecule, or a nucleic acid grafted on an NP) and cause a loss of targeting and/or an increased clearance of ADA–drug complexes, which may lead to suboptimal exposure and loss of efficacy. Therefore, ADAs represent a real challenge in pharmacology as they affect pharmacokinetics, patient safety, and treatment efficacy. Special attention should be given to this troublemaker, especially as the person being treated has a defective immune system that is difficult to control.
5. Perspectives and limitations
NP-based delivery systems hold immense promise for targeted drug delivery and enhanced therapeutic efficacy. However, their approval for clinical use is fraught with significant challenges. It is well known that NP physico-chemical properties influence their behavior in vivo and specifically their bio–nano interactions such as protein opsonization, crossing barriers, and entering the target organ and cells. In all these steps, any unpredicted interaction could lead to unintended toxic effects. In principle, each pathway is regulated by a constant rate. Therefore, knowing the constants, NPs should be designed in accordance for better endocytosis and clearance [120]. However, one primary concern is related to the long-term toxicity of NPs when they are not rapidly excreted. Commonly, the renal clearance of NPs is very efficient and fast with NPs smaller than 10 nm, while limited clearance occurs with larger NPs (∼100 nm). Despite the huge advances in using different carriers, the nanomedicine field is still lacking in a consensual understanding on how nanomaterials interact with human cells, particularly immune cells to improve their biocompatibility and safety [17].
Targeting the desired cells is another clinical issue. Nowadays, most of the NPs approved for cancer reduce the toxicological profile of drug agents rather than enhance the drug therapeutic efficacy. Poor delivery is one of the problems for translating nanomedicine [121]. Wilhelm et al. showed that <1% of NPs reach the tumor site [122] and similarly, it was demonstrated that mRNA encapsulated LNPs are internalized by only 2% of T-cells [123]. To improve the selectivity, NP surface decoration is the main strategy. However, some considerations have been reported. The first one is related to the protein corona formation, which can mask the ligands attached to the NP surface, decreasing the binding with its receptor [124]. Second, the number and the right orientation of appendix moieties can affect receptor recognition [125, 126]. Another central aspect that should be considered is scaling up production. Complex multifunctional formulations require multistep validations of the single components, which is acceptable in a research lab but difficult to maintain in industrial settings [123]. This can result in batch-to-batch variability, impacting both the safety and efficacy of the final product. Scaling up from a pilot plan to large-scale production remains a significant milestone for any manufacturing company and developers. Finally, to be clinically translated, NP syntheses and all their components (external and internal molecules) should be prepared in good manufacturing practice conditions, extending the production period of registered clinical batches and increasing exponentially their cost.
Regarding ATMP monitoring, the prospects are mainly in terms of improving their safety, with the hope that the dose reduction through quantification will also lead to lower costs. Currently, these costs are manageable only for patients/healthcare systems that can afford them. Unfortunately, these treatments are not available to everyone. These two parameters (improved safety and reduced costs) are in line with value-based healthcare, a patient-centered approach to healthcare delivery (https://gestions-hospitalieres.fr/value-based-healthcare/). It aims to improve the health outcomes that matter most to patients—and reducing side effects is certainly one of them—throughout their care pathway, while optimizing healthcare resources and the cost to society. Philosophical value and cost containment are both important, but improving health outcomes is essential to creating value in a holistic, systemic approach.
The main limitation of all these tracking and/or theranostic tools remains on the side of regulatory affairs: how to qualify an ATMP tracking tool, and how to qualify the combination of a tracking tool being internalized in an innovative therapy? What is the qualification for a magnetic hyperthermia therapy derived from an external stimulus internalized in a cargo cell, which can itself interact with its environment?
In terms of perspectives in the theranostic area, the difficulty of active targeting tends toward “cellular nanotheranostics” [127], that is, the use of cargo cells to deliver theranostic NPs in sufficient quantity for a long-term effect coupled with a drastic reduction in side effects.
6. Conclusion
In this review, we presented an overview about NPs, their types, synthesis, characterization, physico-chemical properties, and potential applications in health and diseases. Through different selected examples, we described some successful results in different areas, such as drug delivery, cell therapy tracking, and theranostics. This review is not exhaustive; it covers only a small part of the field. Our aim was not only to point out the success of NP-based strategies, especially very recent ones, but also to insist on some concerns that persist, especially due to the versatility and flexibility of NPs, which is a strong point but can also be a difficult aspect to manage for specific applications. Some aspects of safety were also illustrated. After several decades of research and development, the field of NPs is still constantly evolving. NPs continue to possess tremendous potential for innovation and societal impact. There is no doubt that in the very near future, unsuspected developments of NPs will emerge with the advent and widespread use of new technologies, particularly in the field of miniaturization and implants, microfluidic and flow chemistry technologies, and intradermal, intranasal, and cerebral administration, to cite just a few.
Declaration of interests
The authors do not work for, advise, own shares in, or receive funds from any organization that could benefit from this article, and have declared no affiliations other than their research organizations.
Acknowledgments
DFF would like to thank the Centre National de la Recherche Scientifique (CNRS), the University of Strasbourg (UNISTRA), and all the historical supports of the research carried out at the Institut de Physique et Chimie des Matériaux de Strasbourg, namely, the Région Grand Est, the Agence Nationale pour la Recherche (ANR), Interreg IV Rhin Supérieur, and the Labex Nanoparticles in Interaction with their Environment (NIE). SM acknowledges the support of CNRS, the University of Strasbourg Institute for Advanced Study (USIAS), the Interdisciplinary Thematic Institute IMS, as part of the ITI 2021-2028 program of UNISTRA, CNRS and Inserm (ANR-10-IDEX-0002), and SFRI (STRAT’US project, ANR-20-SFRI-0012), the French National Agency for research (ANR-21-CE17-0026), the European Union’s Regional Development Fund in the context of the Interreg V Upper Rhine program, the FHU ARRIMAGE, and the OMAGE projects funded by Region Grand-Est and FEDER.
1 There are two types of relaxation time: T1, longitudinal relaxation time (in the order of a second) and T2, transverse relaxation time (expressed in milliseconds). In MRI, T1 signal is characterized by the time it takes for hydrogen atoms to return to 63% of their equilibrium position at the end of a pulse.