1 Introduction
The global significance of the chemical interaction of seawater with oceanic crust was first recognized by Hart [33] and Thompson [73], who showed that alteration of basalts by seawater at low temperatures takes up significant amounts of alkalis and that this could exert an important control on the composition of the oceans. Later Wolery and Sleep [80] and Humphris and Thompson [40] showed that higher temperature hydrothermal alteration could also play a major role in controlling ocean chemistry (e.g., uptake of Mg and release of alkalis by the crust). Finally, the discovery of hot springs at the Galapagos spreading center further revealed the influence of high temperature hydrothermal reactions on seawater chemistry (e.g., release of alkalis, silica, and metals, and uptake of Mg by the crust) [24].
Since these groundbreaking studies, numerous hot springs have been sampled along the global mid-ocean ridge system revealing a range of fluid compositions and hydrothermal processes [75]. Fluids have also been sampled from low-temperature ridge flank systems [79], and many studies of oceanic rocks altered at high and low temperatures have been carried out (see reviews in [1,3,29,45]). The global fluxes of heat and mass from submarine hydrothermal systems have thus been significantly revised, but the early examples cited above point out two basic approaches that can be used. A geophysical approach can be taken, using estimates of the amount of hydrothermal heat transport coupled with measured compositions of hydrothermal vent fluids to obtain global fluxes. A mass balance approach can also be taken, using the compositions of altered rocks if their abundance and distribution in the crust can be determined reliably. The problem here is that heterogeneities exist in altered rocks, on the scale of centimeters to hundreds of meters (e.g., [8,15,30,38]), making them extremely useful for understanding hydrothermal processes and the structure of submarine hydrothermal systems, but complicating mass balance calculations by making it difficult to determine what is representative of the crust. Ultimately, however, these two approaches must come to agreement.
2 The structure of submarine hydrothermal systems
Heat flow measured on the seafloor falls below that predicted by conductively cooling plate models out to an average age of 65 Ma, indicating significant loss of heat by convection of seawater through the crust (Fig. 1). Lister [48] classified convection in ocean crust into two types, active and passive. Active systems at mid-ocean ridges (MOR) are driven by a magmatic heat source advected into the crust, and reach high temperatures, 350–400 °C. Hydrothermal alteration occurs predominantly in the sheeted dike complex and plutonic section. Passive systems on ridge flanks are driven by heat conducted into the crust and occur at much lower temperatures (0–150 °C), mainly in the volcanic section of the crust. The reader is referred to the review by J. Honnorez in this issue, and to recent papers [1,3,29,45,60] for details of the mineralogy, chemistry, and processes of hydrothermally altered oceanic crust, which are outside the scope of this paper.
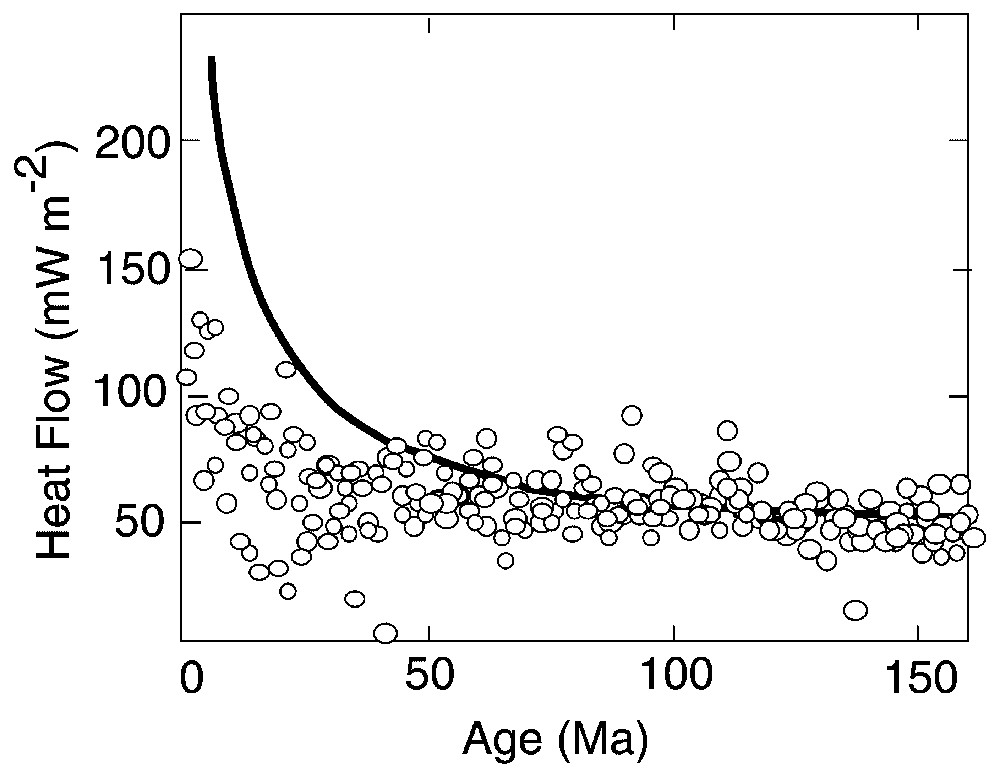
Oceanic heat flow versus age of crust. Measured values (open circles) fall below theoretical conductive cooling curve (heavy line) indicating removal of heat by circulating seawater. Measured values are averages over 2 Ma intervals for Pacific, Atlantic, and Indian oceans (after [70]).
Flux de chaleur océanique en fonction de l'âge de la croûte. Les valeurs mesurées (cercles blancs) tombent sous la courbe théorique de refroidissement conducteur (ligne épaisse noire), indiquant l'évacuation de chaleur par les circulations d'eau de mer. Les valeurs mesurées sont des moyennes obtenues sur des intervalles de 2 Ma, pour les océans Pacifique, Atlantique et Indien (d'après [70]).
3 Hydrothermal fluxes at MOR
3.1 Hydrothermal heat flux at MOR
From the difference between measured heat flow and that predicted by conductive plate cooling models, Stein and Stein [70] calculate a total oceanic hydrothermal heat flux of 11 TW (11×1012 W), or about one-third of the total predicted oceanic heat flux. Using the same approach, Pelayo et al. [55] binned heat flow data into different ages, and calculated an axial hydrothermal heat flux of 0.2 TW for crust less than 0.1 My. This estimate is based on heat flow measurements at MOR, which are problematic, as these require the presence of sediment but the crust is young with little or no sediment cover.
Because of hydrothermal cooling, axial melt lenses lie at crustal depths greater than predicted by conductive cooling models [20]. Morton and Sleep [49] and Sleep [63] used constraints from the depth of assumed steady-state axial melt lenses and models for hydrothermal cooling to obtain an axial hydrothermal heat flux of 1 TW. Using similar models for crustal generation with a dike or melt lens heat source and hydrothermal cooling, Chen and Phipps Morgan [20] calculate a hydrothermal heat flux of 0.9 TW for hydrothermal cooling of new crust out to 1 km from the axis. Baker et al. [13] showed that the incidence of hydrothermal plumes and the heat output from hydrothermal cooling are functions of spreading rate. For a 58 500 km length of spreading ridge (including back-arc basins), this yields a total hydrothermal heat flux of 0.88 sTW.
3He is primordial and its presence in the oceans reflects degassing from basaltic magmas and crystallization of oceanic crust. Jenkins et al. [41] first used the 3He/heat ratio of hydrothermal vent fluids and the excess 3He budget of the oceans in order to calculate global fluxes of hydrothermal heat and fluids. As more vents and plumes were sampled, however, a large variability in the 3He/heat ratio of fluids became evident. Heat fluxes calculated in this way are 1–6 TW and are summarized by Elderfield and Schultz [26].
In another approach, the amount of magmatic heat available from production of new oceanic crust to drive hydrothermal circulation at ridge axes can be calculated [26,42,51,52]. There are two sources of heat to consider: the latent heat of crystallization of basaltic magma, and cooling of crystalline rock from magmatic temperatures (∼1200 °C) to temperatures typical of axial hydrothermal fluids (∼350 °C). Mottl [51] gives an excellent summary of this calculation, including discussion of assumptions and uncertainties for data used in the calculation, as well as a critique of previous calculations. His results are summarized here. Lavas are rapidly cooled by overlying seawater, so the latent heat available from the underlying 5.8 km thickness of sheeted dikes and gabbros is 1.15 ± 0.15 TW. Cooling of these rocks to a hydrothermal temperature of 350 ± 30 °C yields 1.68 ± 0.27 TW, for a total heat flux of 2.83 ± 0.31 TW. This value is an upper limit, as this requires cooling of the entire crust down to the Moho to hydrothermal temperature (∼350 °C). This is clearly unreasonable, as the melt lens that underlies the sheeted dikes would limit hydrothermal penetration within ∼1 km of the spreading axis. Mottl [51] calculates a lower limit for heat output assuming extraction of latent heat from the entire crustal section but cooling only of the sheeted dikes to 350 °C, giving 1.5 ± 0.18 TW. He then gives a best estimate of hydrothermal power output at MOR of 1.8 ± 0.3 TW, by assuming deeper crustal cooling at slow- than at fast-spreading ridges.
Using a similar approach, Elderfield and Schultz [26] calculate values of 2–4 TW. These values are high, however, because they assume hydrothermal cooling of 5–7 km of crust to 350 °C at the spreading axis.
3.2 Hydrothermal fluid flux at MOR
Kadko et al. [42] and Elderfield and Schultz [26] took the heat flux to calculate an axial hydrothermal fluid flux, using the estimated change in water temperature (350 °C) and the heat capacity for water. The latter value is critical, as heat capacity varies with temperature, pressure, and composition, and increases drastically above about 350 °C for 3.5 wt% NaCl solution [14]. Elderfield and Schultz [26] calculate an axial hydrothermal fluid flux of 3–6×1016 g/yr, assuming cooling of the entire crust to 350 °C. If only latent heat is lost, this flux is reduced to 0.7–2.3×1016 g/yr. Mottl [51] integrated heat capacity against temperature in order to more accurately represent heat transport, and obtained a similar hydrothermal fluid flux of 3.07 ± 0.49 to 5.87±0.79×1016 g/yr. His best estimate of hydrothermal heat transport (1.8 ± 0.3 TW) yields a fluid flux of 3.70±0.51×1016 g/yr.
From heat flow measurements and models, Pelayo et al. [55] calculate a fluid flux of 0.4×1016 g/yr on crust less than 0.1 Myr old. Baker et al. [13] calculate an axial hydrothermal water flux of 1.8×1016 g/yr based on hydrothermal plume heat output. Fluid fluxes calculated from 3He/heat ratios are 0.9–5.4×1016 g/yr (summarized in [26]). These are similar to the geophysical estimates above, but the uncertainties are large because of the large range in 3He/heat of vent fluids and in the He contents of submarine basalts. Fluid fluxes can also be calculated from the Sr budget for the oceans, but the 87Sr/86Sr of the ocean is not at steady state and in particular, uncertainties in river inputs result in large errors (see summary in [26]). Currently the best estimates of fluid flux at MOR are derived from the geophysical constraints on the heat budget for formation of ocean crust.
Mottl [51] points out the critical problem that it is not known how much the cooling of lower crustal gabbros contributes to heat and fluid fluxes at spreading axes, and that this is a major uncertainty in estimation of chemical fluxes at MOR. The most intense alteration in oceanic crust occurs in the lowermost dikes and uppermost gabbros at the spreading axis, whereas the significantly diminished access of fluids to greater depths occurs off axis after the melt lens has crystallized [6,8,21,28]. Oxygen and strontium isotopic profiles through the Oman ophiolite indicate significant seawater exchange down to the mantle but other ophiolites (e.g., Troodos) look more like ocean crust, suggesting that cooling of the lower crust at the axis is limited (see discussion in [6]).
3.3 Axial chemical fluxes from heat and fluid fluxes
Many hydrothermal vents having a range of fluid compositions have been sampled along the worldwide MOR system (see summary in [75]). Variations in composition mainly reflect the effects of phase separation and segregation and/or recombination of phase-separated fluids in the subsurface [25]. If all fluids ultimately exit the crust (i.e., no fluid is left behind in the crust), then the extremes of composition should cancel out. The compositions of vent fluids can be combined with the fluid fluxes calculated above in order to obtain chemical fluxes at MOR [26,42,51]. Table 1 shows chemical fluxes at MOR calculated using this approach. These data indicate that hydrothermal systems at MOR are significant sources of alkalis, Be, Ca, Ba, B, Si, H2S, 18O, metals and volatiles, and are sinks for Mg, SO4, alkalinity, and P. In comparison, hydrothermal fluxes first calculated from the Galapagos hot spring data and using 3He/heat ratios were much higher and suggested that MOR hydrothermal systems could account for all the river input of Mg to the oceans [24,76]. These estimates were excessive, however, as they required a source of alkalis greater than that contained within the entire oceanic crust. According to the estimate in Table 1, MOR hydrothermal systems can provide a sink for only 30% of the river input of Mg, the implication being that the remainder must be taken up on ridge flanks [52]. Other hydrothermal processes are also important, however, in determining the net effect on crustal and oceanic concentrations of these elements.
Estimates of global hydrothermal fluxes
Estimation des flux hydrothermaux globaux
Axial fluxes | Flank fluxes | Seawater | River flux | ||||||
(mol/kg) | (1010 mol/yr) | ||||||||
Element | Conc. hydroth. | Hydrothermal flux | Mass balance | Hole | Sites | Conc. warm | Max. warm | ||
fluid (mol/kg) | (1010 mol/yr) (a) | from rocks (c) | 504B | 417/418 (j) | spring (k) | spring flux (k) | |||
(1010 mol/yr) | (1010 mol/yr) | (1010 mol/yr) | (mol/kg) | (1010 mol/yr) | |||||
Li | 1.4 – 4.8 | 0.3–0.8 (d) | =−0.2 (d) | 9 μ | −0.18 | 26 m | 1.4 | ||
K | 28 – 85 | 0.008 (e) | −6.5 (h) | −40 to −49 | 6.88 m | −33 | 9.8 m | 190 | |
Rb | 0.032 – 0.116 | −0.0038 | 1.12 μ | −0.0026 | 1.3 m | 0.037 | |||
Cs | 0.00035 – 0.0007 | −0.0004 | 2 n | 0.00048 | |||||
U | −0.0004 | ||||||||
Be | 0 | 0.0037 | |||||||
Mg | 0 | −169 | −0.07 (e) | −30.8 (h) | 17 to −26 | 0.98 m | −540 | 53 m | 530 |
Ca | 1.1 – 160 | 20 (e) | 2.9 (h) | 140 to −140 | 55.2 m | 470 | 10.2 m | 1200 | |
Sr | 87 μ | 0 | 110 μ | 0.25 | 87 u | 2.2 | |||
Ba | >8>42.6 μ | 0.029 – 0.16 | 0.14 μ | 1 | |||||
SO4 | −103 | −6.8 (f) | 7.72 (f) | 17.8 m | −110 | 28 m | 370 | ||
Alk | −8.8 – −12 | 0.43 m | 2.3 m | 3000 | |||||
Si | 53 – 81 | 490 (e) | −31 (h) | −71 | 360 m | 1.8 | 0.05 m | 640 | |
P | 0.5 μ | −0.0055 | −0.16 | 0.3 μ | −0.026 | 2 μ | 3.3 | ||
B | 0.14 – 0.55 | 570 μ | 1.7 | 416 μ | 5.4 | ||||
Al | 0.015 – 0.074 | 0.02 μ | 6 | ||||||
Mn | 1.4 – 4.2 | −0.23 (h) | 0.3 | 7.8 μ | 0.052 | 0 | 0.49 | ||
Fe | 2.8 – 23 | −18 (h) | −1 | <0.1 μ | 0 | 0 | 2.3 | ||
Co | 0.03 n | 0.011 | |||||||
Cu | 0.036 – 0.16 | 0.01 μ | 0.5 | ||||||
Zn | 0.14 – 0.39 | 0.01 μ | 1.4 | ||||||
Ag | 0.02 n | 0.0088 | |||||||
Pb | 0.01 n | 0.015 | |||||||
As | 27 n | 0.072 | |||||||
Se | 2.5 n | 0.0079 | |||||||
CO2 | 12.3 – 148 | 150–240 (i) | −260 | −16 | 2.3 m | ||||
CH4 | 0.82 – 3 | 0 | |||||||
H2 | 0.4 – 1.8 | 0 | |||||||
H2S | 10 – 120 | 1.3 (f) | 0 | ||||||
H2O | −2800 (g) | −330 (h) | −560 (b) | ||||||
18O | 0.28 (b) | −0.1 (b) |
Metals and sulfide are precipitated as sulfide minerals in massive sulfide deposits, in the black ‘smoke’ from hydrothermal vents, or in the subsurface as high temperature fluids mix with seawater to form diffuse low-temperature venting [39]. The latter process comprises up to 90% of the axial heat and fluid fluxes [32,56,58] so much of the metals and sulfide mobilized from the crust at depth is retained in the crust [2,81]. Oxidation of sulfides, iron and manganese in hydrothermal plumes and the overlying water column, subsequent scavenging of other elements (REE, P, As, V, U, Cr, Pb) within plumes, and deposition of these particulates in sediments return much of this material to the crust (see review in [43]). One third of the river flux of sulfate is taken up by the crust in MOR systems (Table 1). This occurs by precipitation of anhydrite, which may be dissolved at lower temperatures and returned to the oceans [2]. More recently, Alt et al. [11] document the occurrence of gypsum in the Macquarie Island Ophiolite, and suggest that significant amounts of anhydrite may be preserved in the crust at low temperatures, implying that this could be an important sink for sulfur [11]. These authors provide no estimates of global chemical fluxes, however. Teagle et al. [72] suggest that anhydrite may not form in the amounts predicted by the calculations in Table 1, but their estimate is based on drillcore samples that are plagued by low core recovery, so it is possible that the abundance of anhydrite in the drillcore may not be representative.
Sulfur isotopic evidence indicates that some seawater sulfate is reduced to sulfide and combined with primary igneous sulfide leached from the crust to produce the sulfide source to the oceans and result in the formation of sulfide deposits at axial vents [2,61]. Table 1 shows a wide range of axial sulfide fluxes from vent fluid data, but this sulfide source to the oceans is most likely near the smaller end of the range.
Low temperature alteration of the volcanic section on ridge flanks is also an important complement to the hydrothermal alteration at ridge axes. The alkali and 18O sources at MOR are essentially offset by the alkali and 18O sinks on ridge flanks, and much of the calcium and carbon released at MOR may be accounted for by secondary calcite formed at low temperatures in the volcanic section (see below).
3.4 Axial chemical fluxes from rock chemistry and mass balance
Calculation of chemical fluxes based on mass balance and chemical analysis of rocks is complicated by the heterogeneity of alteration effects and the problem of reliably determining what is representative of the crust. Early studies of pillow basalts suggested that if 10–30% of the crust was altered to chlorite-rich metabasalt the resultant sink of Mg and source of Si and Ca to the oceans would equal the river fluxes of these elements [33,40]. Other early rock-based estimates of the Mg sink and Ca source from high temperature axial alteration are much lower, however [50,74]. These early studies all based their results on analyses of altered pillow basalts, whereas it later became apparent that most hydrothermal alteration occurs in the sheeted dikes and uppermost gabbros [8,10,28,30,31]. Estimates from Hole 504B (Table 1) that take into account estimates of vein mineral abundances indicate that alteration of the sheeted dikes is a small source of K and sink for Mg. The source of Li from alteration of sheeted dikes based on Hole 504B is low compared to that based on heat flux, in part because the rocks from Hole 504B have primary compositions that are highly depleted in incompatible elements, so their low primary Li contents limit the amount of Li that can be leached from the rocks [19]. The small sulfate sink in the crust is present as anhydrite, mainly in veins. The rock estimate yields a small H2S sink in the rocks, in contrast to the H2S source based on heat flux because sulfur lost from dikes and gabbros is retained in mineralized zones within the crust [2]. The Si source estimated from the altered rocks is greater than that from the heat balance. Although the uncertainties are large, these data agree with the suggestion that most of the Mg balance may be taken up in ridge flanks [52]. The Mg and sulfate estimates from Hole 504B may be low, however, because of loss of more altered breccias during drilling. Recovery in the hydrothermally altered transition zone was ∼30% and in the dikes only ∼15%. Interpillow areas and dike margin breccias gain significant Mg in chlorite cements, but are preferentially ground up during drilling and not recovered. A significant proportion of the sulfide and Li lost from the crust is released from the uppermost plutonic section, and other alkalis may similarly be liberated from these rocks.
Laverne et al. [47] calculate even smaller chemical fluxes from the Hole 504B sheeted dike section than those in Table 1. These authors did not include veins or breccias in their calculations, however, nor did they consider the significant problem of loss of material during drilling. Talbi et al. [71] calculate global fluxes for some elements during hydrothermal alteration of gabbros, and suggest that these rocks may be sources of Si and Ca and sinks of Fe and Mg. The same problems of relating a few dredged samples to the entire crust remain, however. Other studies of bulk changes in hydrothermally altered gabbros indicate little chemical change other than Sr and O isotopic effects [37] and the previously mentioned S and Li changes. More data for distributions of alteration types and vein minerals, and for chemical changes in oceanic sheeted dike complexes and plutonic sections are clearly needed in order to better estimate chemical fluxes from mass balances like this.
Several hydrothermal systems and deposits hosted in peridotite have recently been discovered and appear to be common on slow spreading centers [23,44,46]. Not taken into account in Table 1 are chemical fluxes associated with serpentinization of peridotites on the seafloor. Wallmann [78] suggests that the amount of water taken up by serpentinization may be comparable to the uptake by hydrothermally altered sheeted dikes and gabbros, essentially doubling this flux in Table 1. Alt and Shanks [7] make a similar inference about sulfur in serpentinites, that the potential sulfur sink in serpentinized oceanic mantle (1.3–19×1010 mol/yr) may be comparable in magnitude to the sink of seawater sulfur in the crust and the source of basaltic sulfur to seawater that occur in combined axial and flank systems. Fluxes of methane and hydrogen from these peridotite-hosted systems may be significant, but remain unquantified.
4 Hydrothermal fluxes on ridge flanks
4.1 Heat and mass fluxes on ridge flanks from hot spring data
Mottl's [51] best estimate of hydrothermal heat transport for active MOR systems (1.8 ± 0.3 TW) is only about 15% of the total oceanic hydrothermal heat flux of 11 TW [70], so the major portion of hydrothermal heat flux occurs in passive systems on ridge flanks. The fluid flux in these systems depends on the temperature at which circulation occurs. Oxygen isotopic data for secondary phyllosilicate, carbonate, and silica minerals in seafloor basalts from various sites indicate temperatures of 0–150 °C (see review in [3]), a somewhat larger range than the temperatures measured in oceanic crust where flank convection is still active (2–90 °C; [52]). Mottl and Wheat [52] divided flank systems into cooler (<25 °C) systems, which advect large amounts of heat and have little change in the composition of circulating seawater fluids (< 10% Mg loss), and warmer (>45 °C) systems, where most heat is lost by conduction and fluids lose most (>80%) of their Mg. Recent work on warm springs and basement waters from the Juan de Fuca Ridge has shown linear correlations between temperature and fluid composition (for Mg, Ca, SO4, K, 87Sr/86Sr) from 15–64 °C [27,79]. Over this temperature range therefore, global flank fluxes could be determined if the total power output on ridge flanks could be defined. In order to constrain the maximum fluxes for such warm ridge flank environments Wheat and Mottl [79] balanced the river input of Mg to the oceans by uptake in warm (60 °C) flank systems, then used this fluid flux and the composition of the warm spring fluids to calculate maximum fluxes for other elements (Table 1). Chemical fluxes for this environment are minor for most elements, except for Mg, K, Ca, SO4, and B, which all exceed 25% of the river flux. Low sulfate concentrations in the warm basement waters are interpreted to reflect sulfate reduction in sediments [79], so the sulfate sink does not represent basement reactions. However, Alt et al. [11] suggest that sulfate reduction may also occur in basement. For two ODP sites on the Juan de Fuca flank that show minor sulfate depletion in basement waters there is no sulfur isotopic fractionation in sulfate [27], which is not consistent with the implied microbial sulfate reduction. Butterfield et al. [18] show that flank circulation having Δ(87Sr/86Sr)/heat ratios only one third to one half that found in the Juan de Fuca flank fluids can balance river and axial hydrothermal Sr inputs to the oceans to give the observed temporal increase in 87Sr/86Sr of the oceans (0.000 054 per million years).
For some elements (e.g., Mg, Ca, B) the maximum warm flank fluxes are in the same direction and similar in magnitude to those at higher temperatures in the axial systems (Table 1). For others, however, fluxes from the flank systems partly to totally offset the fluxes at ridge crests: e.g., the maximum sinks for K and C on flanks are similar to the sources of these elements at ridge axes. The flank Li and Rb sinks calculated this way, however, are much smaller than the axial source fluxes.
The heat flux calculated for warm flank circulation is only about 15% of the total advective heat loss on ridge flanks, so the remaining ∼85% of the heat loss must take place at lower temperatures (<25 °C) on ridge flanks [52,79]. At a temperature of 10 °C this fluid flux would be more than 36 times greater than the maximum warm flank fluid flux. Chemical changes for seawater fluids in such very low temperature systems are largely unknown, but because of the very large fluid fluxes even small (∼1%) changes in fluid composition would be significant [79]. Such very low temperature systems may account for further uptake of alkalis, which may balance the axial fluxes for Rb and Li and, as suggested from rock data, the crust may be a net sink for these elements overall (see below). Similarly, the later formation of secondary calcite in basalts at low temperatures during aging of the crust can account for Ca lost on the flanks and even result in a net uptake of Ca by the upper crust, and can enhance the C uptake (see below; [5,67]).
4.2 Ridge flank chemical fluxes from rock chemistry
Low-temperature alteration on ridge flanks is essentially restricted to the volcanic section (e.g., see reviews in [1,3,29]). As in axial hydrothermal systems, if the chemical changes in altered crust can be determined reliably then these can be used to infer chemical fluxes during ridge flank alteration.
Early estimates of global chemical fluxes were based mainly on the chemistry of bulk pillow basalts dredged from the seafloor [33,73]. Later studies took into account variations with depth in the crust using data from lavas sampled by deep ocean drilling (e.g., [22,34,66,74]). Subsequent studies have tried to better estimate the composition of the uppermost crust by accounting for different rock and alteration types, and also include vein minerals, which can significantly affect mass balances [4,9,67–69].
This has been done in two ways. One way is to measure the abundances of all rock and alteration types and veins in drillcores and combine chemical analyses of these in a mass balance (e.g., [5,9,12]). Another approach is to combine powders of different rock and alteration types, including veins, in representative proportions to make composite samples of altered crust, and then analyze these to get the bulk composition of the upper crust [62,64,65,68,69].
Examples of results of these approaches are given in Table 1. Alt et al. [9] combined measurements and analyses of cores in a mass balance to obtain chemical fluxes for the volcanic sections of ODP Holes 504B and 896A. Those results are combined in Table 1: the upper 300 m of the volcanic sections of the two holes are averaged and combined with the 250 m lower volcanic section from Hole 504B. The Hole 504B flank fluxes are generally small compared to high-temperature axial fluxes. If the Li sink in the mineralized transition zone in Hole 504B (0.09×1010 mol/yr) is factored in, the Li sink in the volcanic section essentially balances the high-temperature Li source in the dikes and gabbros [19]. In contrast, earlier estimates suggest that the Li flux from axial high temperature alteration of deeper crust exceeds the low temperature sink for Li in the lavas, resulting in a net Li flux to the oceans [59]. The Ca flux from Hole 504B volcanic section is small in part because Ca lost from basalts is offset by the formation of calcite in veins. The small Mg sink resides in smectite veins and breccia cements. The net uptake of iron by the section may be real, and is related to the formation of hydrothermal celadonite at low temperatures, from distal hydrothermal fluids derived from high temperature reactions at depth in axial systems (see review in [3]). Loss of sulfur occurs as the result of degassing during eruption and by oxidation of rocks at low temperature [2].
The small chemical fluxes calculated for Hole 504B probably result mainly from loss of material during drilling. Core recovery in the volcanic section was only about 30%, and more intensely altered breccias, interpillow materials, and veins are lost during drilling. Geophysical and geochemical logs indicate that the abundance of breccias in the basement may be more than twice that recovered in the drillcore [16,17]. Dating of vein minerals indicates that most alteration is complete within about 10–15 Ma [35,36,66,77], although carbonate formation may continue for much longer [5]. It has thus been argued that because Hole 504B is in relatively young crust (5.9 Ma), basement alteration may not be complete there. Two points suggest that alteration at Site 504 is mostly complete, however: first, the recovered rocks exhibit all the effects seen in the oldest ocean crust [62]; and second, with the exception of the uppermost ∼100 m the basement at Site 504 is already sealed to convection [9,10].
Using the same approach and data for several ODP drill cores, Alt and Teagle [5] showed that the amount of carbon taken up on ridge flanks exceeds that degassed at MOR, and that the crust is a net sink of 1.5–2.4×1012 mol C per year. This is in agreement with a previous estimate by Staudigel et al. [67] using the composite approach on a different sample set.
Table 1 shows fluxes calculated from a ‘super’ composite from DSDP Sites 417 and 418 [65,69]. This is a mixture of rock (pillows and flows) and volcaniclastic (hyaloclastite) composites from several different depth intervals. Most fluxes calculated from the super composite are near zero. Significant errors arise because of the uncertainty in the composition of the fresh protolith, which is calculated from glass and phenocryst compositions and abundances. In particular, variations in the abundances of olivine and plagioclase phenocrysts result in large errors for Ca, Fe, Mg, and Al and fluxes of these elements are zero within the uncertainties (Table 1; [65,69]). These sites had good core recovery (>70%), including highly altered interpillow volcaniclastic materials, but the composites contain veins in unknown proportions leading to further uncertainty. From a comparison of geophysical and geochemical logs with core analyses, Revillon et al. [57] suggest that composites for 165 Ma Site 801 in the Pacific overestimate alteration somewhat. Alt [4] points out that although the composites for Site 801 take into account results from geophysical logging in order to estimate the abundances of different rock types, the volcaniclastic (breccia) material that was mixed into the Site 801 composites is biased toward interflow material (sediment). In contrast, the breccias documented by the logs at this site are mainly highly fractured pillows and thin flows cemented by secondary minerals. Thus, while 30% breccia determined from logging is reasonable for the Site 801 basement section, the amount of sediment mixed into the composites is too great leading to greater apparent alteration as estimated by the composites. This points out again the problem of heterogeneity and a need to understand in detail the distribution of alteration types and veins in order to mix these in proportions representative of the crust.
Because of the low concentrations of U, Rb, and Cs in fresh rocks, the fluxes of these elements as determined from the Site 417/418 composites are more reliable than those of other elements. A significant amount of U is transferred to the upper crust in ridge flanks, and the Cs uptake offsets that released in high-temperature axial systems. The K sink from the composite is larger than that calculated from mass balance at Site 504, and is essentially equivalent to the K source at MOR. Hart and Staudigel [34] suggest that the uptake of K, Rb, and Cs by the upper crust via low temperature processes exceeds their loss from the lower crust during high-temperature axial processes, so the crust is a net sink for these elements. B behaves similarly to the alkalis, with the low temperature uptake of B exceeding the high temperature hydrothermal source of B to the oceans [64].
Part of the chemical differences between flux estimates from individual sites may be the result of heterogeneity of alteration effects. Both the composite approach and the mass balance approach, however, are affected by the same problem: heterogeneity of rock types and alteration. The published fluxes based on mass balance (e.g., Site 504) may underestimate alteration because of loss of material during drilling, but this problem can be overcome by incorporating data from geophysical logging of drillholes (see discussion in [4]). For one example of the composite approach (Site 801), it is suggested that this overestimates alteration effects because the rock samples incorporated into the composites were not truly representative of the basement section [4,57]. The composites at Site 417/418 used some information from vein counts, but quantitative data from detailed logging of vein minerals and alteration effects in the entire drillcore, like those from Site 801, were not available. These estimates thus have a greater uncertainty than the more recent estimates from Site 801, which can be corrected for the sediment excess. Clearly, the much older Site 417/418 basement contains a greater amount of carbonate veins and thus has higher C and Ca contents, leading to greater or net uptake of these elements compared to the much younger Site 504/896 basement.
In contrast to the axial 18O source to the oceans, 18O is taken up at low temperature on ridge flanks [54,78]. Wallmann's [78] estimate for 18O cycling indicates an imbalance between sinks for 18O in ridge flanks and continental weathering and the axial hydrothermal 18O source, suggesting that the δ18O of seawater has increased over the Phanerozoic. In contrast, other recent estimates suggest that these processes are in balance, and that the oceans have maintained their δ18O within about 1 per mil throughout the Phanerozoic [53].
5 Summary
Heat and fluid fluxes in high temperature axial systems are best determined by geophysical methods, which can then be combined with vent fluid compositions to derive chemical fluxes. Axial hydrothermal systems at MOR are significant sources of alkalis, Be, Ca, Ba, B, Si, 18O, metals and volatiles, and are sinks for Mg, SO4, alkalinity, and P. Chemical fluxes calculated by mass balance using data from hydrothermally altered rocks sampled by deep ocean drilling are generally small, probably because of loss of the most altered material during drilling. Additional data for sheeted dike complexes and plutonic sections are needed to better constrain chemical fluxes from rock data. Most of the hydrothermal heat anomaly in ocean crust occurs at low temperatures in off-axis flank systems. Flank processes enhance the axial sources of some elements (e.g., Mg, Ca, B, 87Sr/86Sr) but are sinks for others (e.g., alkalis, C, 18O), offsetting the axial fluxes of these elements. Chemical fluxes for flank systems determined from rock chemistry and mass balance are better than those for axial systems, but errors still arise from heterogeneities and the need to reliably determine what is representative of the basement.
Acknowledgements
The author thanks Jose Honnorez and Olivier Rouxel for helpful reviews.
Preparation of this manuscript was supported by NSF Ocean Sciences.