Version française abrégée
Le diamant et son polymorphe la lonsdaléite sont les deux matériaux naturels les plus durs connus à ce jour [9]. La recherche de matériaux plus durs reste un défi de la physique des matériaux et se fait, soit au travers d'expériences à ultra-hautes pressions, soit par des simulations numériques [10,21,23,35]. Aucune de ces approches n'a mis clairement en évidence de formes cristallines du carbone stables à très haute pression et température, autres que le diamant et la lonsdaléite [20,21,28].
Des diamants formés lors de processus de choc ont été décrits dans des roches de nombreux cratères d'impacts terrestres [1,8,13–17,22,26]. Des formes amorphes et peut-être cristallines du carbone sont fréquemment associées à ces diamants, mais leurs natures et leurs mécanismes de formation restent obscurs. Nous présentons dans ce qui suit une description des différentes phases du carbone observées dans les gneiss choqués du cratère d'impact de Popigai, en Russie. Pour cela, nous avons utilisé la spectroscopie Raman, le rayonnement synchrotron pour la micro-diffraction et la micro-fluorescence des RX, la microscopie électronique par balayage et l'observation en lumière naturelle réfléchie de surfaces polies de roches. Les trois premières techniques permettent une caractérisation de la structure et de la chimie des phases à une échelle spatiale de quelques microns. La dernière permet de comparer la dureté relative des minéraux par rapport à la dureté du matériau utilisé pour le polissage.
Les observations en microscopie électronique à balayage montrent, dans les gneiss de Popigai, des pseudomorphoses de cristaux de graphite. Ces zones de carbone pur sont polycristallines. La Fig. 1 présente des observations en lumière naturelle pour différentes focalisations d'une telle zone dans un gneiss poli avec de la pâte diamantée. On y observe l'association de différentes formes du carbone ne présentant pas le même relief (la même hauteur) par rapport aux grains minéraux les entourant (quartz, cristobalite et plagioclase). On trouve, à l'intérieur, des pseudomorphoses des zones (70 μm de long) formant une marche positive de 10 μm par rapport à l'ensemble des autres grains de carbone et des minéraux entourant la pseudomorphose. Cette observation suggère la coexistence, au sein de la pseudomorphose, de phases minérales du carbone ayant des duretés différentes, certaines d'entre elles plus dures que le diamant utilisé pour le polissage.
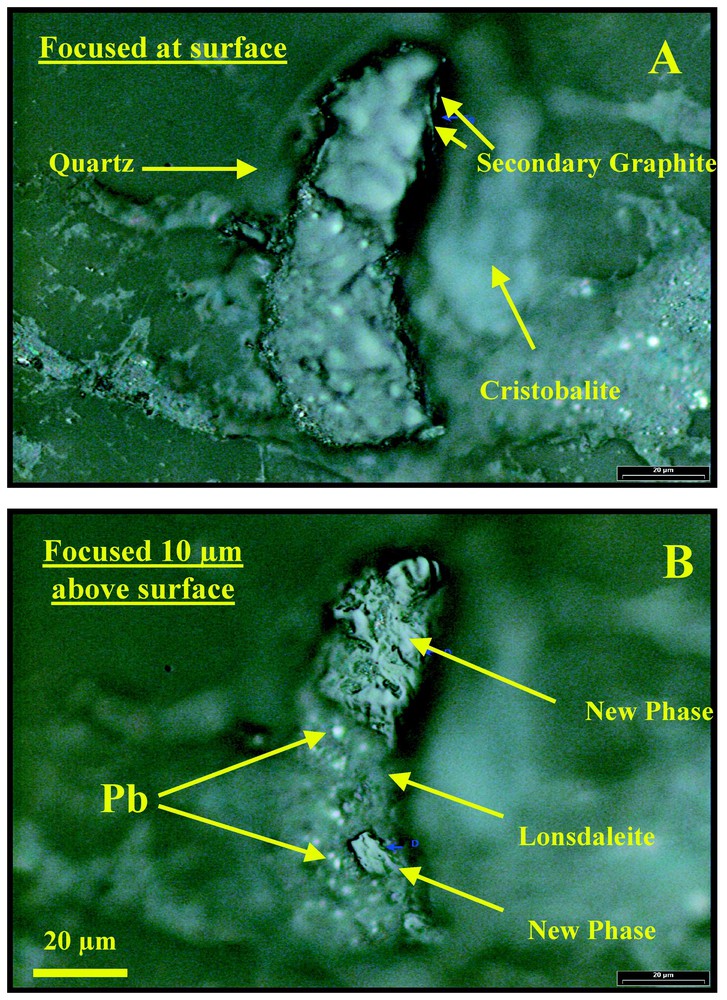
Reflected light photographs of carbon composite grain #17 from a polished section of the shocked gneiss in Popigai (A) focused at the surface of the polished thin section showing the hard high relief part out of focus, (B) photograph focused 10 μm above the surface, thus depicting the surface features of the new carbon phase with gouges and metallic lead oxide (Pb; arrow) scraped from the polishing disc.
Photographies en lumière réfléchie des grains de carbone composites d'une section polie des gneiss de Popigai. (A) Mise au point à la surface de la section polie montrant une zone en relief (floue), (B) la même zone, avec une mise au point 10 μm au-dessus de la surface de la section polie ; on observe la texture de la surface de la nouvelle phase du carbone avec des rayures et des traces d'oxyde de plomb arraché au disque de polissage.
Différentes techniques ont été utilisées pour déterminer la nature cristalline et la structure des différentes phases du carbone présentes dans les pseudomorphoses de graphite. La microspectroscopie Raman révèle, dans les zones sans relief, la présence de lonsdaléite avec une bande caractéristique à 1325 cm−1, une fréquence légèrement plus basse que celle du diamant à 1332 cm−1. On trouve aussi, sur les bords, des pseudomorphoses du graphite ordonné, avec sa bande caractéristique vers 1585 cm−1. Les zones en relief présentent un spectre avec deux bandes larges (Fig. 2) à 1390 et 1600 cm−1, qui ne correspond à aucun spectre Raman connu des phases amorphes ou cristallines du carbone. Les bandes caractéristiques de PbO [7] sont aussi observées dans cette zone en relief. Cette phase résulte de l'oxydation de copeaux de plomb arrachés au disque de polissage pendant la phase terminale de préparation de la section polie.
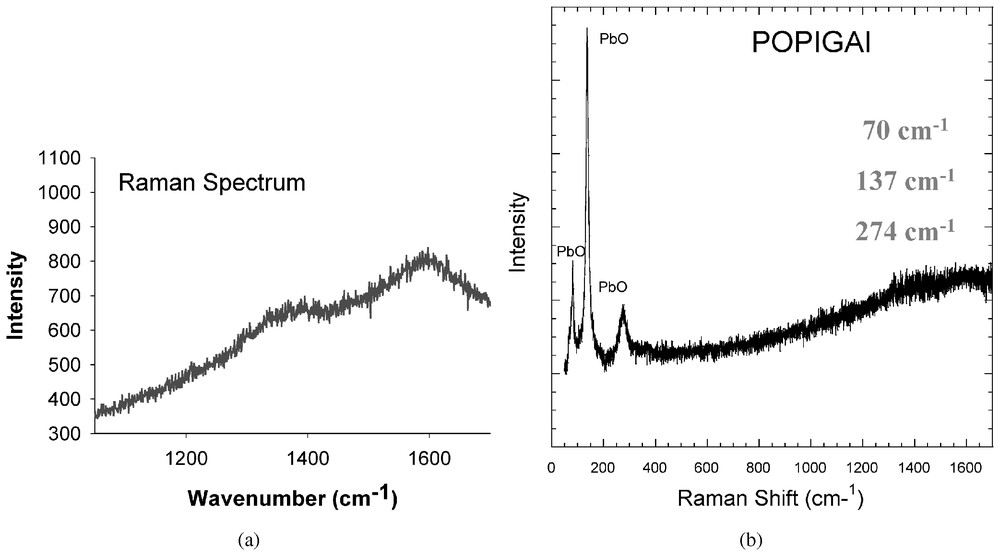
(a) First-order Raman spectra acquired at the high relief portion of the composite grain (Fig. 1b; new phase) depicting the two broad Raman bands. (b) Low-frequency part of the Raman spectrum depicting the three characteristic bands of PbO at 70, 137 and 274 cm−1, given as inset on the right side of the figure, respectively.
(a) Spectre Raman du premier ordre enregistré sur la zone en relief de la Fig. 1b (new phase). On observe deux bandes larges. (b) Partie basse fréquence du spectre Raman de la même zone montrant les modes de vibration caractéristique de PbO à 70, 137 et 274 cm−1.
La cartographie par diffraction et fluorescence des RX à une échelle de quelques micromètres montre une association concentrique remarquable de phases de carbone pur (Fig. 3), de graphite et de lonsdaléite, ainsi que d'une structure cristalline du carbone inconnue, confirmant les mesures par spectroscopie Raman. La phase du carbone faisant relief sur la section polie est entourée de lonsdaléite et possède un spectre de diffraction ne correspondant à aucune phase cristalline répertoriée du carbone et à aucune des autres phases minérales présentes dans la roche. Le spectre de diffraction de cette phase (Fig. 4 et Tableau 1) correspond à celui d'une phase cubique avec un paramètre de maille de 14,697(1) Å et un groupe d'espace Pm3m. L'allongement des taches de diffraction indique que les zones à lonsdaléite et à nouvelle phase sont formées de cristallites de petites tailles (40 nm) présentant une forte orientation préférentielle. Cette observation est en accord avec la formation de ces phases par un processus de choc violent et bref. La texture ne permet pas d'affiner la position des atomes de carbone dans la nouvelle phase et donc de calculer sa densité. Notons finalement que le spectre Raman présenté sur la Fig. 2 correspond probablement à du carbone amorphe entourant les cristaux de la nouvelle phase.
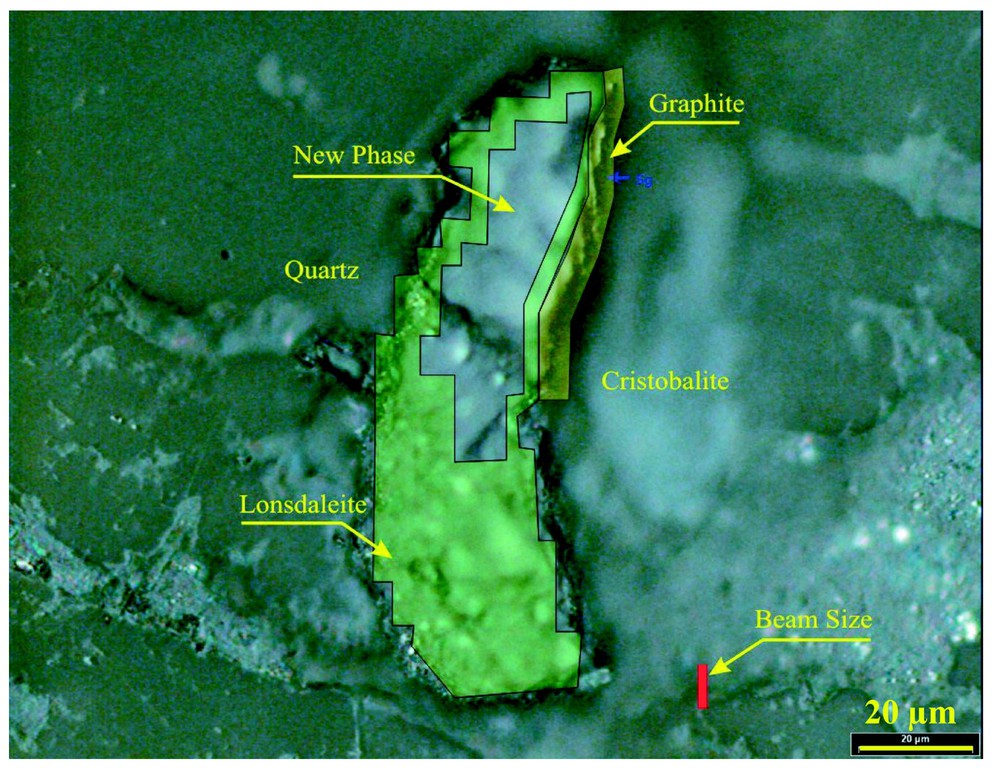
Results of the synchrotron X-ray diffraction, imaging and X-ray fluorescence fine-scale mapping laid out on the microscopic photograph of grain #17 shown in Fig. 1a, depicting the spatial settings of the various carbon phases. The figure shows also the size of the synchrotron beam (lower right, red) for comparison.
Superposition sur la photographie de la Fig. 1 des résultats de diffraction des RX, d'imagerie et de fluorescence X. On observe ainsi l'agencement spatial des différentes phases du carbone. La taille du faisceau de RX de la source synchrotron est indiquée en bas à droite, en rouge.
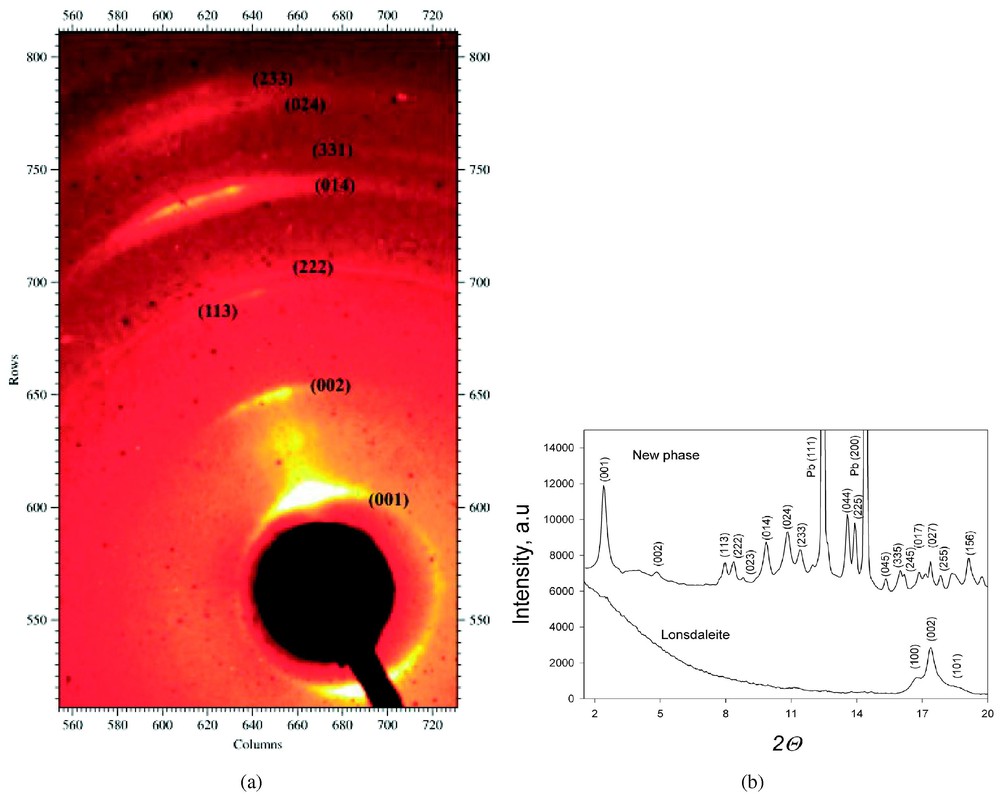
X-ray diffraction pattern of the new carbon phase. (a) Diffraction picture of the new carbon polymorph demonstrating the preferred orientation due to shock-induced dynamic deformation (streaking of the diffraction spots). (b) The full X-ray patterns of the new phase along with the strongest lines of metallic lead (Pb (111) and Pb (200)) reflections (upper panel) and lonsdaleite (lower panel). The high background of continuum in the lonsdaleite pattern at 2θ<11° is due to the presence of an amorphous carbon phase.
Diagramme de diffraction de la nouvelle phase du carbone. (a) Image de diffraction du nouveau polymorphe, montrant l'orientation préférentielle des grains (tâches étirées). (b) Spectre de diffraction complet de la nouvelle phase du carbone. On observe des pics intenses dus à la présence de plomb (Pb (111) et Pb (200)) et de lonsdaléite. Le fond continu important à 2θ<11° est dû à la présence d'une phase amorphe du carbone.
Indexed peaks of the X-ray diffraction pattern and Miller indices collected from the new super-hard natural polymorph of carbon from Popigai in the assemblage shown in Figs. 1 and 3, respectively. The structure is cubic with a=14.697(1) Å and space group Pm3m
Indexation du spectre de diffraction et indices de Miller de la phase ultra-dure du carbone des gneiss de Popigai. La zone d'étude correspond à celle des Figs. 1 et 3. La structure est cubique avec a=14,697(1) Å et Pm3m comme groupe d'espace
dobs (Å) | dcalc (Å) | Intensity (%) | h | k | l |
14.6991 | 14.6985 | 100 | 1 | 0 | 0 |
7.3479 | 7.3493 | 6 | 2 | 0 | 0 |
4.6478 | 4.6481 | 2 | 3 | 1 | 0 |
4.4333 | 4.4318 | 26 | 3 | 1 | 1 |
4.2470 | 4.2431 | 28 | 2 | 2 | 2 |
4.0762 | 4.0766 | 3 | 3 | 2 | 0 |
3.6707 | 3.6746 | 23 | 4 | 0 | 0 |
3.5671 | 3.5649 | 23 | 4 | 0 | 1 |
3.3700 | 3.3721 | 7 | 3 | 3 | 1 |
3.2876 | 3.2867 | 49 | 4 | 2 | 0 |
3.1348 | 3.1337 | 26 | 3 | 3 | 2 |
2.9390 | 2.9397 | 3 | 5 | 0 | 0 |
2.8579∗ | Pb | 1 | 1 | 1 | |
2.5982 | 2.5984 | 44 | 4 | 4 | 0 |
2.5580 | 2.5587 | 38 | 2 | 2 | 5 |
2.4751∗ | Pb | 2 | 0 | 0 | |
2.2949 | 2.2955 | 13 | 0 | 4 | 5 |
2.2414 | 2.2415 | 24 | 5 | 3 | 3 |
2.2151 | 2.2159 | 8 | 6 | 2 | 2 |
2.1922 | 2.1911 | 8 | 5 | 4 | 2 |
2.1210 | 2.1215 | 17 | 4 | 4 | 4 |
2.0780 | 2.0887 | 15 | 1 | 0 | 7 |
2.0186 | 2.0190 | 22 | 2 | 0 | 7 |
1.9990 | 2.0002 | 14 | 2 | 5 | 5 |
1.8663 | 1.8667 | 27 | 1 | 5 | 6 |
∗ Reflections from Pb metal particles scraped from the polishing disc.
L'ensemble de ces observations permet de conclure que le processus de choc dans les gneiss de Popigai a conduit à la formation d'une forme cristallisée du carbone de structure cubique plus dur que la lonsdaléite et donc que le diamant. Pour le moment, il est impossible de savoir si cette nouvelle espèce minérale s'est formée lors du maximum de pression et de température subi par la roche lors du choc ou si elle résulte de la transformation d'une phase stable à plus haute pression et température lors de la décompression. Ce nouveau minéral n'a jamais été synthétisé en laboratoire ou produit lors de simulation numérique de type ab initio. Il pourrait être à l'origine du carbone amorphe fréquemment observé en intercroissance avec des diamants dans différentes structures d'impact à la surface de la Terre [14,16].
1 Introduction
Cubic diamond (space group Fd3m) and hexagonal lonsdaleite (space group P63/mmc) are the hardest natural materials known [9]. However, a search for phases with even greater hardness is continuously conducted by ultra-high pressures experiments and theoretical calculations [10,21,23,35]. The mechanism of static and dynamic graphite to diamond phase transformation has been the subject of extensive experimental investigations, however details of transition path are not fully clarified [3–6,11,12,29–31,34]. Ab initio molecular dynamics calculations give more insight in details of the possible inversion process [27]. The inversion of graphite to diamond or lonsdaleite may take place in a multi-stage process [28]. The process includes a pressure-induced sliding of the graphite atom planes towards orthorhombic stacking, from which a fast structure collapse to both hexagonal and/or cubic diamond takes place [28]. Graphite-diamond phase transformation is reconstructive and requires change of the bonding type from sp2 to sp3. Although the graphite–diamond phase boundary is considered to be fairly well established [2], there are still considerable uncertainties as to the lonsdaleite stability field, the diamond/lonsdaleite phase boundary and the behaviour of carbon at ultra-high pressures and temperatures, in particular the expected transition to a theoretically predicted metallic state with 6 coordination [28,35]. The theoretical data also predict the existence of other dense carbon polymorphs [35]. Experimental investigations at pressures between 150 and 200 GPa showed remarkable changes in the Raman spectra of diamond anvils [21]. The results were interpreted as indicating that considerable changes in the bonding properties and structure of diamond occur under these ultra high-pressure conditions [21]. Investigations at pressures to 320 GPa using diamond as internal pressure standard revealed, however, no evidence of any transitions in diamond [20].
Shock-induced diamonds were reported from several impact craters [1,8,13–17,22,26]. Some of the impact diamonds are associated with dense amorphous carbon, thought to have formed by melting of the diamond in the hot impact breccia [17]. Yet, the origin of this amorphous carbon phase is far from clarified. Carbon phases encountered in the polished sections from the shocked Popigai gneiss were investigated using reflected light microscopy, laser microRaman spectroscopy and microbeam synchrotron radiation.
2 Experimental techniques
2.1 Laser microRaman spectroscopy
Raman spectra were recorded with a DILOR XY and a LABRAM spectrometer equipped with confocal optics and a nitrogen-cooled CCD detector at the ‘École normale supérieure de Lyon’, France. A microscope is used to focus the excitation laser beam to a 2-μm spot and to collect the Raman signal in the backscattered direction. Three excitation wavelengths were used: 488, 514 and 650 nm. The laser power at the sample was 2 to 50 mW to avoid deterioration of the sample.
2.2 Synchrotron X-ray diffraction, fluorescence and imaging
The grain in the Popigai sample #17 (Fig. 1) was thereafter cored out using a high-precision micro-drilling instrument and mounted on a hole in a metal plate holder for X-ray studies. Imaging, X-ray diffraction and X-ray fluorescence studies were carried out at ID22 beam line at the European Synchrotron Radiation Facility (ESRF), Grenoble, France. The ID22 beam line is dedicated to fluorescence, imaging and diffraction with micron-size analysis capabilities. The wavelength of the incoming X-ray beam was selected to 0.62 Å (equivalent to 20 keV), with a relative bandwidth of 10−4. The monochromatic 20-keV X-rays were focused by a set of 100 parabolic Al Compound Refractive Lenses; the beam intensity delivered was approximately 5×109 photons s−1, with a spot-size of about 2×4 μm on the sample [18]. A PIN diode detector, operating in the current integration mode, was positioned before the sample to monitor the flux of the incident focused beam. The sample in the metallic holder was positioned in the image plane on a high precision XYZ remote-controlled stage, and aligned along the X-ray path. A ∅100-mm CCD camera located behind the sample recorded WAXS diffraction images simultaneously with the fluorescence measurements. The X-ray microbeam was scanned over the whole carbon grain and the surrounding silicate matrix. Diffraction, fluorescence data and imaging information were collected for every beam spot. X-ray fluorescence was collected at the front-side of the cell with a Si (Li) solid-state diode detector of 150-eV resolution at 5.89 keV, set at 165° from the incident beam in the horizontal plane. The detector, placed 3 cm away from the cell, was mounted on motorized translation stages and shielded with a collimator (∅=4 mm) in order to reduce the Compton scattering collection and to improve the fluorescence/scattering ratio. X-ray diffraction and fluorescence phase identification mapping was laid out on the microscopic photograph (Fig. 1), thus allowing fine-scale spatial phase separation and further delineation of the textural relations of the coexisting phases.
3 Results
We investigated impact diamond bearing shocked gneisses from the Popigai crater, Russia. Polished sections of shocked gneisses contain transparent multiphase carbon platelets, each displaying considerably variable relief. The relief varies within individual multiphase grains between 5 and 12 μm. This is strongly suggestive of the presence of different coexisting transparent materials with different polishing hardness. A large (70 μm) transparent platelet with very high relief on one side (height up to 10 μm above the section surface; Fig. 1) was selected for the Raman and X-ray powder diffraction and fluorescence synchrotron studies. The part with highest relief displays convex and uneven surface with rough gouges (Fig. 1b). Metallic lead scraped from the lead polishing disc due to high resistance to polishing was also encountered on the surface of the grain, thus indicating its extreme high polishing hardness (Fig. 1). The 0.25-μm diamond powder used for polishing did not succeed in cleanly producing a flat polished surface and erase scratches and gouges formed during cutting of the section slab (Fig. 1b). This indicates the enormous difference in hardness between the polishing diamond powder and the natural high-relief carbon material. Energy Dispersive X-ray (EDX) fine-scale mapping analysis at low voltage without carbon coating with a Field Emission Scanning Electron Microscope (FESEM) indicated that the entire grain is pure carbon.
We conducted several successive experiments to determine the crystalline nature of the various carbon components of this grain: (1) laser microRaman spectroscopy and (2) fine scale micro-beam synchrotron imaging, X-ray diffraction and X-ray fluorescence investigations. Raman spectrum measured at the area with highest relief revealed two broad Raman Bands at ∼1390 cm−1 and 1600 cm−1 (Fig. 2a) and high-fluorescence background. The characteristic one-phonon band of diamond at 1331 cm−1, the graphite main band at 1580 cm−1 and the disorder band of graphite (‘D’ band) at 1355 cm−1 [19,32,33] are absent. Three sharp bands in the low frequency region at the wave numbers 70, 137 and 274 cm−1, respectively (Fig. 2b) were also found. These bands are characteristic of orthorhombic PbO [7]. They originate from oxidized very small lead filings scraped during the final polishing on the lead disc. The broad Raman bands are different from spectra of nanometer-sized diamond [37], diamond-like amorphous carbon films, amorphous diamond [24,36] or any other carbon polymorph [19]. Small flakes (up to 7 μm) of polycrystalline secondary graphite as evidenced also from the absence of the ‘D’ Raman band at 1355 cm−1 diagnostic of deformed graphite constitute the outer most portion of the platelet. These graphite flakes probably formed by back transformation of diamond, lonsdaleite, or other dense carbon phase. Raman spectra diagnostic of lonsdaleite are also observed in normal relief zones within this complex carbon aggregate.
The fine scale X-ray diffraction and fluorescence mapping revealed a complex structured carbon grain (Fig. 3). The platelet is encompassed by a 40-μm long secondary polycrystalline cristobalite on the right side and by a 15-μm long undeformed secondary quartz on the left both formed by back transformation from a parental dense silica polymorph at high post-shock temperatures that melted the matrix plagioclase (Fig. 3). The carbon phases and the surrounding low-pressure silica are enclosed in wavy plagioclase quenched from the gneiss melt. The point imaging indicated that the transparent interior with the variable relief is homogeneous, with no silicate or oxide inclusions. The synchrotron fluorescence point mapping revealed no other element heavier than carbon (except lead metal scraped from the polishing disc). This result confirms the pure carbon nature obtained by EDX analysis. X-ray diffraction mapping of the platelet indicated the presence of three distinct crystalline carbon phases in a remarkable concentric shell-like setting (Fig. 3), in addition to an amorphous carbon phase. The portion with the highest relief of 10 μm above the section surface is a new super hard polymorph of carbon (see below). This new phase is enveloped by lonsdaleite (Fig. 3). The outer most 7-μm thick shell (Fig. 3) consists of individual crystallites of secondary 2H graphite (as evidenced from the strongest X-ray reflection at d=3.376 Å). Lonsdaleite occupies the portion of the fairly polished transparent interior of the grain, with a lower relief than that of the new phase, thus manifesting its lower polishing hardness (Figs. 1 and 3). The X-ray pattern of the portion with the highest relief (Fig. 3) is different from that of any known carbon polymorph (Fig. 4). Pattern also contains the (111) and (200) reflections of metallic Pb (Fig. 4, Table 1). The pattern of the new phase is also different from those of any mineral present in the gneiss, like cristobalite, quartz, plagioclase, garnet, rutile, ilmenite, zircon or any lead oxide. Crystal-bond epoxy is not the source of this pattern, because this epoxy is amorphous. Contamination from the neighbouring phases can thus entirely be ruled out. Twenty-three diffraction lines (Fig. 4) were obtained from the super-hard carbon (Table 1). They could be unambiguously indexed in terms of a cubic cell (space group Pm3m) with a=14.697(1) Å; cell volume a3=3174.58 Å3.
The diffraction patterns of lonsdaleite and of the new phase indicate extremely small grain size of the crystallites (<40 nm) of both phases and demonstrate a remarkable high degree of preferred orientation and texturing (Fig. 4a). This is diagnostic of highly shock stressed material and adds ample evidence against contamination by polishing material. Although, for improving diffraction statistics, X-ray patterns were collected from sample oscillated around the ω-axis of the goniometer, the quality of the data is still not sufficient for structural refinement (especially taking into account the relatively large unit cell). Nevertheless, obtained reflections intensities were used in Evandour program [25] with fixed space group Pm3m and variable number of atoms per unit cell for trail structures. However, the diffraction data and the calculated unit cell do not unambiguously allow a calculation of the density.
4 Discussion
The spatial arrangement with the very hard carbon phase occupying the core of the dense carbon platelet with lonsdaleite completely surrounding it may reflect a sharp change in the peak-shock pressures from the interior outwards. We do not know at present whether the new carbon phase is a primary species or a quench phase of an unknown metastable even denser carbon polymorph. However, this arrangement may hint that the new phase emerged during decompression from a denser and metastable carbon polymorph. The difference in polishing hardness between lonsdaleite and the new species cannot have resulted from difference in the grain size, since the X-ray diffraction lines of both species show similar structures indicative of extremely small grain size and similar structuring and preferred orientation due to shock deformation (Fig. 4a). Raman spectra obtained for the studied grains are suggestive of the presence of additional material with both sp3 and sp2 bonding and high fluorescence. The spectrum probably originated from an amorphous carbon that masked the Raman spectrum of the new species due to the high-fluorescence background.
We do not know whether the new phase is a stable polymorph with a distinct P and T stability field in the carbon phase diagram. The fine-grained preferred orientation negates the formation of the new species via shock-induced intermediate amorphisation of a parental carbon polymorph that in turn revealed the formation of the new phase after decompression. A carbon polymorph with similar properties or crystal structure was not synthesized at high pressures and temperatures so far. To the best of our knowledge, such polymorph was not predicted by theoretical calculations either. Further experimental studies may reveal the synthesis of this phase with extraordinary properties. It remains to be seen whether the amorphous carbon phase previously encountered inter-grown with impact diamond in several impact structures [16,17] and with lonsdaleite in this sample is produced by amorphisation of this very hard carbon species.
Acknowledgements
The authors are grateful to Gilles Montagnac (UMR 5570 CNRS) for help during Raman measurements and to Friedrich Seifert (Bayerische Geoinstitut, Bayreuth) for critically reading the manuscript before submission.
They are also grateful to Glaf Medenbach (Universität Bochum) for cleanly coring out the sample out of the section.