1 Setting the scene
This brief paper does not attempt to provide an exhaustive summary of the work of many Australian scientists on this topic. It provides illustrations taken from the author's research of the effects of climate change on regolith materials. Southwestern Australia is unusual in that it has experienced a prolonged period of subaerial weathering during leaching conditions which has been followed by the imposition of an arid climate under which large amounts of salts have accumulated in the regolith. This peculiar confluence of a deep lateritic regolith permeated by complex saline solutions, has resulted in the generation of the unusual mineralogical and geochemical phenomena that are the main subject of this paper. These materials and processes are not solely of scientific interest but are also of great concern to managers of agricultural and urban land and water who are combating development of soil salinity, sodicity, acidity and water logging due to inappropriate management of this complex hydrogeochemical system.
The major geological feature of southwestern Australia is the Yilgarn Craton, an Archaean complex of igneous and metamorphic rocks that has mostly been exposed to aerial weathering since the mid-Proterozoic (Fig. 1) [1]. The region has been tectonically stable for much of this period so that the landscape is extensively peneplained and mantled by deep regolith. A major regolith material is the lateritic profile that formed by in situ largely isovolumetric weathering and which is of common occurrence in tropical regions [13]. The laterite profile contains several zones or horizons with the clay rich saprolite and overlying plasmic zones commonly comprising much of the profile which can extend to depths of several tens of metres (Fig. 2) [14]. These materials are sometimes described as the pallid zone of the lateritic profile [4]. The near surface zones of lateritic profiles commonly exhibit evidence of disturbance, addition or erosion of materials but most of the landscape is mantled by the in situ clay-rich laterite zones that have commonly developed from igneous and metamorphic rocks.

Periods of exposure to subaerial weathering in southwestern Australia. Modified from [1].
Période d'exposition à l'altération sub-aérienne dans le Sud-Ouest australien (d'après [1]).

A schematic lateritic regolith profile. Modified from [4] and [14].
Profil schématique de régolithe latéritique d'après [4,14].
A consequence of extensive isovolumetric chemical weathering is that the fabric of clay-rich laterite profile materials is often extremely open and highly porous (Fig. 3). Clay crystals are packed together in diverse arrangements reflecting the extent to which topotactic/epitactic alteration of primary minerals has preserved parent rock and mineral fabrics [5]. For example, single crystals of muscovite may have altered to tightly packed stacks of kaolinite crystals with little porosity [15], whereas feldspars and micas are commonly replaced by highly porous, randomly oriented clay mineral assemblages, generally consisting of admixtures of platy kaolinite and tubular halloysite (Fig. 3) [2]. In this micrograph, crystals of kaolinite appear to be altering to tubular halloysite by a rolling process. Many of these materials have a total porosity of about 50% [12], which may be visualised as a 20-m thick zone of regolith containing a 10-m thick void and it is this void volume that contains ground waters with their large and complex salt solutions.

Transmission electron micrograph of an ultra-thin section of highly porous halloysite (marked 2, 3, 4)/kaolin (marked 1) lateritic saprolite from southwestern Australia. Adapted from [16].
Photo de microscopie électronique à transmission d'une section ultra-fine d'halloysite (points 2, 3, 4)/kaolinite (point 1) d'une saprolite latéritique très poreuse du Sud-Ouest australien (adapté de [16]).
In order to interpret the influence of this large pore volume on the storage and mobility of water and dissolved salts it is necessary to consider the pore size distribution of the materials. An approximate rule of thumb, used by soil physicists [20], is that water flows under the force of gravity through pores larger than about 100 μm. Water is retained by capillary forces in pores with diameters between about 0.5–100 μm and this water can be extracted by plants. Water in smaller pores is extracted with great difficulty and this problem is exacerbated by osmotic effects if the water is saline. Water retained in pores smaller than about 0.5 μm cannot be removed by plants so that removal of dissolved ions in this water is only possible by diffusion of the ions and the extreme tortuosity of small pores makes diffusion a very slow process [20]. When we examine the pore size distribution of typical plasmic and saprolitic materials (the laterite pallid zone of [4]) formed on felsic (granite) and mafic (dolerite) igneous rocks), it is evident that most of the pore volume resides in pores smaller than 0.5 μm (Fig. 4) [12]. During the period of lateritic weathering all pores would have contained solutions more nearly approximating the composition of rainwater than the present-day saline solutions. Ions released by exchange at the surfaces of crystals of primary minerals would have slowly diffused through small pores in the clay matrix towards large water filled pores and would then have been removed by leaching. The environmental requirements for continuous operation of this process are: (1) medium–high rainfall which is in excess of evapotranspiration by vegetation, leading to a saturated regolith for at least some of the year; (2) an effective internal and external drainage systems to remove dissolved ions and (3) sufficient time for the weathering process to achieve complete dissolution of the susceptible minerals in the parent material. All three conditions were fulfilled during the Tertiary period in southwestern Australia with external drainage being provided by large and quite slowly draining river systems flowing through broad valleys across the gently sloping landscape.

Water retention characteristics of lateritic pallid zone materials formed from granite and dolerite. The greater porosity of dolerite pallid zone (broken line) is due to the presence of sand-size quartz in granite pallid zone (continuous line). For both materials, most of the pore volume is in pores smaller than 0.5 μm.
Caractéristique de la rétention d'eau des matériaux latéritiques de la zone blanchâtre formés à partir de granite et dolérite. La plus grande porosité de la zone blanchâtre sur dolérite (ligne en tireté) est due à la présence de quartz de granulométrie sableuse dans la zone blanchâtre du granite (ligne continue). Pour les deux matériaux, la plus grande partie du volume poral provient de pores inférieurs à 0,5 μm.
With the onset of arid to semi-arid climates in the Miocene (Fig. 5) [19], the palaeoenvironment on the Yilgarn Craton largely degenerated into zones of intermittently connected drainage sumps which only rarely provided significant external drainage to the oceans or adjacent basins [1]. Consequently, many valleys are occupied by mobile playa lakes with bordering fields of lunate and elongated dunes [9]. Halite, gypsum, calcite, dolomite, sepiolite, palygorskite, smectite, authigenic illite and other less common authigenic minerals (e.g., carnotite) are constituents of playa sediments and downwind valley side soils [7]. However this surface expression of salinity provided by the playa lakes and associated sediments represents only a few percent of the total salt load of the landscape. Much salt (e.g., 2000 t) [3] is present as saline to hypersaline groundwater, pore water and crystalline salt within the highly porous regolith. The composition of these ground waters is variable with the major ion ratios resembling those for seawater which supports the view that the major source of salts has been rainfall [3]. Under native bush much of the rainfall was transpired by plants and very little was available for leaching so that salts have accumulated in the regolith. Within the saline groundwater, the concentration of Si, Al, Fe, H+, other ions and dissolved oxygen are highly variable reflecting inter alia local geology and hydrology. For example, the concentration of dissolved Si may range from 5–130+ mg, with a tendency for dissolved Si to increase with decreasing pH and decreasing total salinity (i.e. decreasing electrical conductivity (Fig. 6) [10].
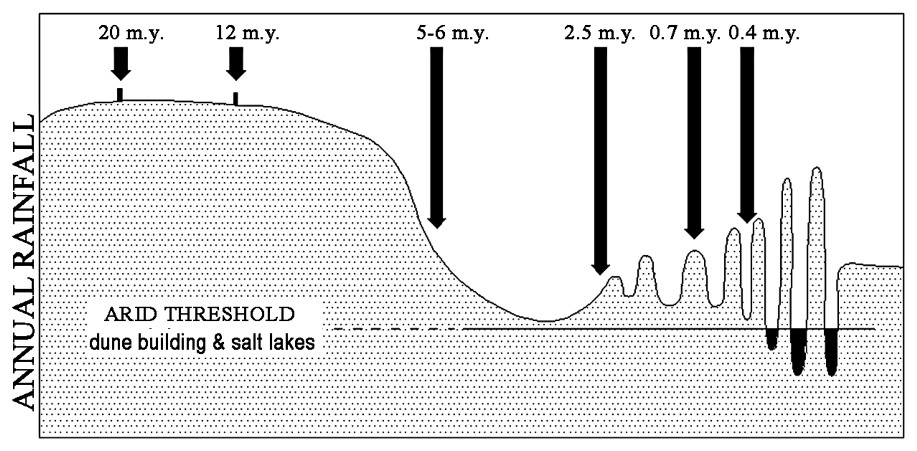
A schematic representation of changes in rainfall in arid to semi-arid areas of southwestern Australia during the last twenty million years. Adapted from [19].
Représentation schématique des variations des précipitations dans les régions arides à semi-arides du Sud-Ouest australien pendant les 20 derniers millions d'années (adapté d'après [19]).

The relationship between pH, total salt concentration (EC) and dissolved Si concentration in groundwaters from southwestern Australia.
Relation entre pH, concentration saline totale (EC) et concentration en silice dissoute dans les eaux souterraines du Sud-Ouest australien.
2 Alteration of regolith
The above overview identifies the fascinating geochemical situation occurring in regolith in southwestern Australia. Laterite materials containing kaolin group minerals and gibbsite that have formed in response to removal of alkalis and silicon from the primary minerals are now impregnated by saline water enriched in those same alkalis and silicon. Interpretation of equilibrium phase diagrams and calculation of chemical equilibrium using programs such as PHREAQ [10] indicate that kaolin and some other lateritic minerals in the regolith are not in geochemical equilibrium with actual solutions. Thus one might anticipate that extensive alteration of lateritic regolith is occurring as it adjusts to its new geochemical environment yet there is little evidence for this in most profiles where kaolin group minerals appear to persist [1,4]. Possibly alteration of kaolin to illite, smectite, alunite, etc. by saline ground waters are very slow processes with surface reactions such as direct incorporation of K and a tetrahedral silica sheet into kaolin structure being improbable so that congruent dissolution is probably an essential intermediary step. Reactions will also be retarded by slow diffusion through submicron pores to and from the reacting surfaces of minerals. Singh and Gilkes [16] have reported on the alteration of platy kaolinite to tubular halloysite in this regolith, which may be a consequence of contact with highly saline ground water as this process can be reproduced experimentally under similar conditions [17].
Although most of the regolith column appears to be unaltered by contemporary groundwater, evidence for neoformation of minerals is more apparent in those parts of the regolith that correspond to present or past groundwater surface levels or near surface soil horizons. In such situations, deposition of opaline silica (silcrete), ferricrete, calcrete and other materials is commonplace (Fig. 7). Opaline silica commonly impregnates the regolith matrix, often completely filling the extensive pore volume that was described earlier. Micrographs of silica impregnated materials shows densification of both the kaolin ground mass and kaolin pseudomorphs after primary mineral grains (Fig. 8). High-resolution electron micrographs of thin sections of silica-impregnated kaolinite clay show that the voids between microfabric kaolin crystals become almost entirely filled by a dense mass of nanometric grains of silica (Fig. 9) [18]. The kaolin crystals contain defects and have serrated ends but these features are possibly original rather than being due to alteration associated with silicification. Electron microprobe analysis (EMPA) of micrometric volumes of silicified kaolinite saprolite has provided a convenient procedure for quantifying the extent of silicification. If all the alumina in the analysed volume is allocated to kaolin, the silica present in kaolin matrix can be calculated and in the absence of other silicates excess silica will be amorphous silica. Typical data for silicified kaolin matrix derived from feldspar and silicified, partly kaolinised muscovite grains in granitic saprolite are shown in Table 1. For the kaolin matrix derived from feldspar about 40% of the material is opaline silica, the small amount of iron can be a structural constituent of kaolin [8] or free iron oxide. The original mica grains (e.g., Fig. 8) would have contained about 10% K2O so that the three altered mica grains analysed (Table 1) contain various concentrations of K2O and represent very different extents of kaolinisation. Furthermore weathered mica grains are commonly exfoliated with the kaolin groundmass invading the interlamella spaces that are created. In this case the concentration of K2O in the grain was used to calculate the proportion of unweathered mica remaining in the analysed volume. It is apparent that the amounts of amorphous silica in the (partly) kaolinised mica grains are much less (9–18%) than in the kaolin matrix (about 40%). This difference is partly due to the topotactic alteration of individual mica to kaolin [15], which creates much less pore volume than the isovolumetric alteration of K-feldspar (the main parent minerals of the matrix).
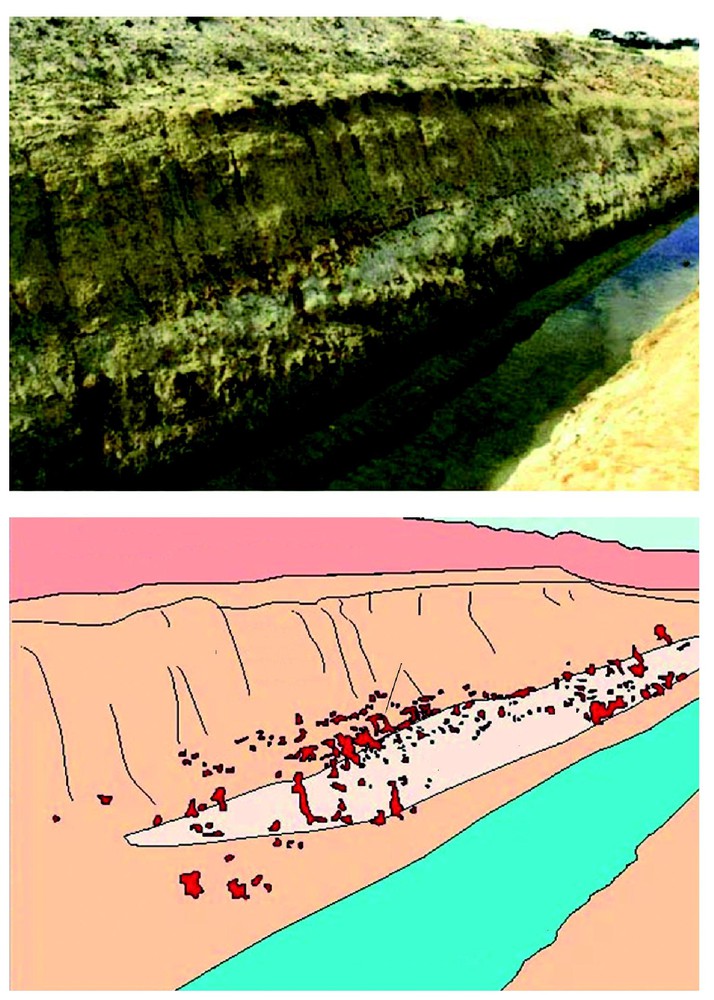
A drain at Narembeen, southwestern Australia showing a lens of silcrete and associated ferricrete in kaolinitic regolith.
Drain à Narembeen (Sud-Ouest australien), montrant une lentille de silcrète associée à un ferricrète dans une régolithe kaolinitique.
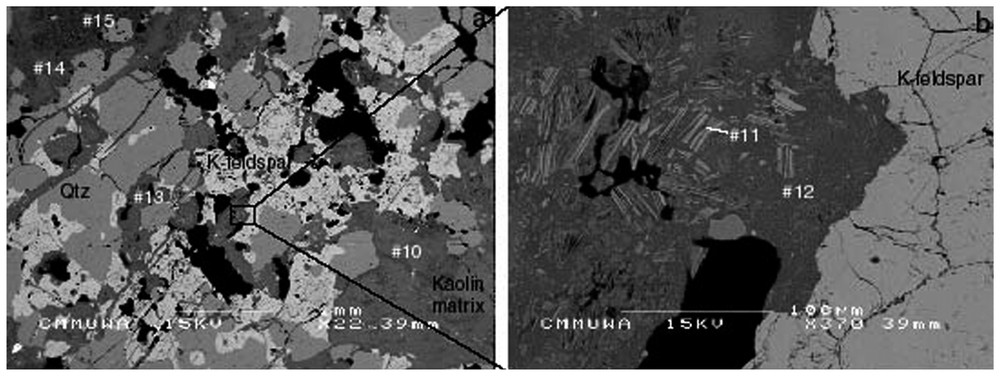
Scanning electron micrograph of a polished section of silicified kaolinitic granite saprolite from Merredin, southwestern Australia. Partly kaolinised, exfoliated mica crystals are present within a kaolin ground mass that has formed by kaolinisation of feldspar. Analyses for some of the indicated points are given in Table 1.
Photo au microscope électronique à balayage d'une section polie d'une saprolite de granite, kaolinitique silicifiée, à Merredin (Sud-Ouest australien). Des cristaux exfoliés de mica, partiellement kaolinisés, sont présents dans un fond matriciel kaolinique, qui s'est formé par kaolinisation du feldspath. Des analyses de certains des points sont reproduites dans le Tableau 1.
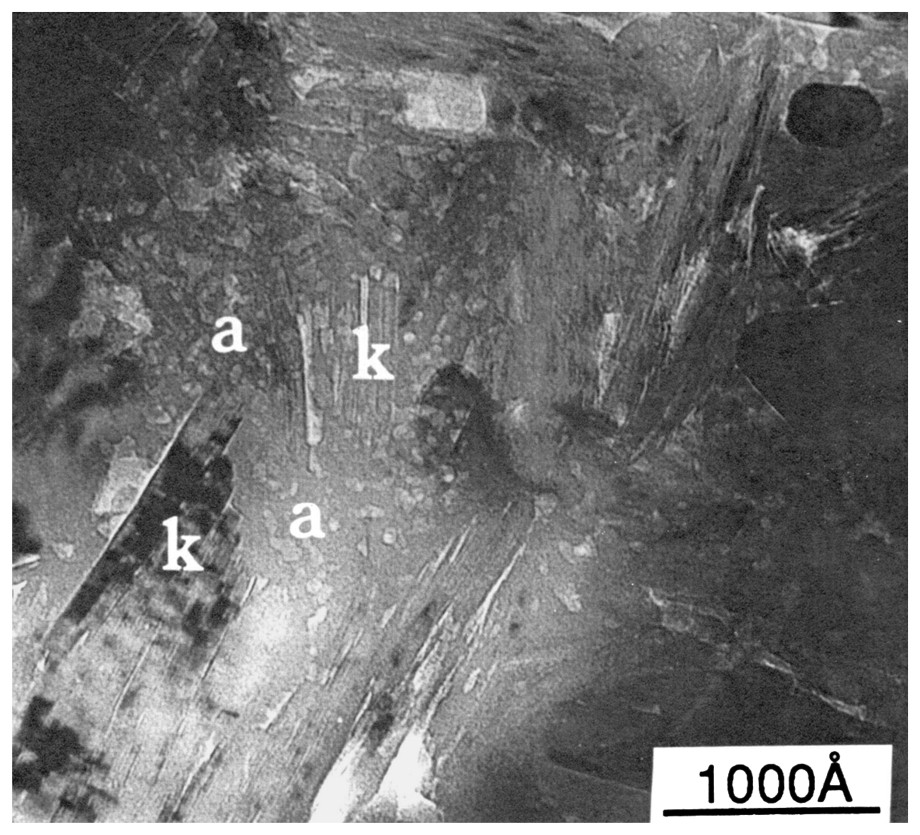
A high-contrast transmission electron micrograph of an ultra-thin section of silicified kaolinitic regolith from southwestern Australia. Composite kaolin crystal(s) are embedded in a matrix of a granular secondary silica (a).
Photo au microscope électronique à transmission à fort contraste d'une section ultra-mince de régolithe kaolinitique silicifiée du Sud-Ouest australien. Des cristaux composites de kaolin sont inclus dans une matrice de silice granulaire secondaire (a).
Analyses by EMPA of micrometric volumes in silicified Merredin granite saprolite. Calculated amounts of amorphous silica are shown for kaolin matrix that has mostly formed from feldspar and for kaolinised mica grains
Analyses APME de volumes micrométriques dans la saprolite silicifiée de granite de Merredin. Les teneurs calculées de silice amorphe sont fournies pour la matrice kaolinique, qui s'est en grande partie formée à partir de felspath, et pour les grains de mica kaolinisés
Description | SiO2 (wt%) | Al2O3 (wt%) | Fe2O3 (wt%) | K2O (wt%) | Amorphous silica (wt%) |
Kaolin matrix | 73.2 | 24.7 | 1.2 | 0.3 | 44 |
Kaolin matrix | 71.0 | 26.8 | 1.1 | 0.1 | 39 |
Kaolin matrix | 71.3 | 27.3 | 1.0 | nd∗ | 39 |
Altered mica | 58.4 | 39.2 | 1.1 | 1.2 | 12 |
Altered mica | 55.4 | 39.2 | 1.9 | 2.0 | 9 |
Altered mica | 54.4 | 31.2 | 5.0 | 8.0 | 18 |
∗ not detected.
Some deposition of opaline silica in the kaolinitic regolith has occurred within a few metres of the soil surface. This zone of the regolith is pervaded by plant roots that extract water, the roots of many plant species exclude silicon and thus enhance deposition of opaline silica in the rhizosphere. This process commonly results in the formation of rhizoconcretions, layers and lenses of silcrete that contain abundant silicified plant roots (Fig. 10). EMPA analyses of silicified plant roots show that in many instances silicified roots consist of almost pure opaline silica (Table 2, mean value, 96.6% SiO2). Lens shaped bodies of silcrete occur in the regolith and may reflect the local effects of water extraction from the vadose zone by single trees or shrubs. A schematic representation is shown in Fig. 11 [10] of silcrete lens formation in the vadose zone in lateritic pallid zone. The silcrete lens formed above the water table and beneath a horizon of lateritic colluvium, which is a common association in southwestern Australia. Silcrete has also formed in seepage zones and other geomorphic settings.

Scanning electron micrograph of a thin section of silcrete from Wundowie, southwestern Australia showing plant roots that have been replaced by secondary silica. Analyses for indicated points are given in Table 2.
Photo au microscope électronique à balayage d'une section fine de silcrète de Wundowie (Sud-Ouest australien), montrant des racines de plantes qui ont été remplacées par de la silice secondaire. Les analyses des points indiqués sont reproduites dans le Tableau 2.
Analyses by EMPA of spots in silicified plant roots from silcrete at Wundowie, southwestern Australia (values in weight %). Spots A and B are shown in the micrograph (Fig. 10)
Analyses APME de taches dans des racines silicifiées de plante du silcrète de Wundowie, Sud-Ouest australien ; les taches A et B sont indiquées sur la micrographie de la Fig. 10
Spot # | SiO2 | Al2O3 | Fe2O3 | Al2O3/SiO2 |
A | 83.36 | 12.88 | 2.3 | 0.15 |
B | 99.58 | nd∗ | nd∗ | 0.00 |
C | 97.83 | 1.25 | 0.48 | 0.01 |
D | 99.53 | 0.23 | 0.00 | 0.00 |
E | 99.56 | 0.18 | 0.26 | 0.00 |
F | 99.79 | 0.12 | 0.00 | 0.00 |
Mean | 96.61 | 2.44 | 0.51 | 0.02 |
∗ not detected.
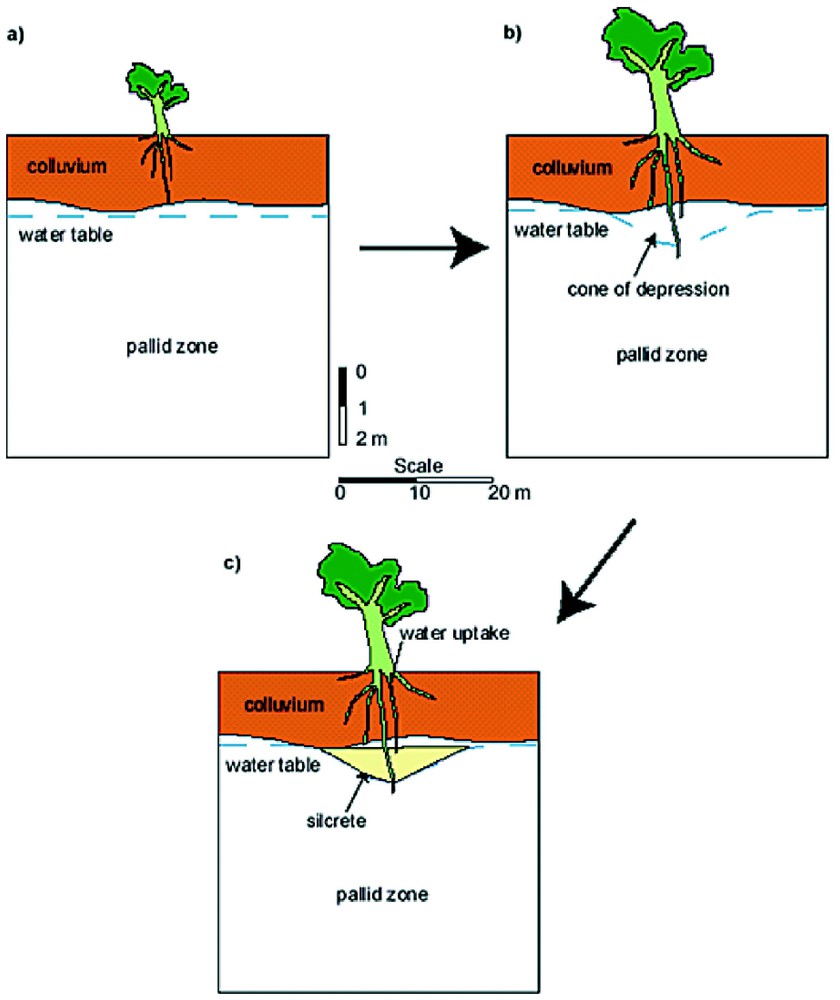
A schematic sectional diagram of lateritic regolith (in situ pallid zone with overlying colluvium) showing how water extraction by tree roots in the vadose zone lowers the local water table and leads to the formation of a lens of silcretised pallid zone.
Coupe schématique d'une régolithe latéritique (zone blanchâtre in situ avec colluvium sus-jacent) montrant combien l'extraction d'eau par les racines d'arbre dans la zone vadose abaisse la nappe locale et conduit à la formation d'une lentille de zone blanchâtre silcrétisée.
The above description has focussed on silcrete formation, as this material is widespread in the region. However many other minerals are deposited from the groundwater within the regolith or at the soil surface. Where groundwater has a suitable composition calcrete/dolocrete is formed in kaolinitic and other regolith by deposition of calcite/dolomite within the porous matrix (Fig. 12). Relic fabric and distinctive features of the lateritic regolith such as in situ kaolinised mica grains have been preserved by induration by carbonates, as is also the case for silicification [5]. Gypsum, iron oxides, authigenic silicates such as smectite and sepiolite and many other minerals may also impregnate lateritic regolith. Illuviation of sodic clay resulting from contact between kaolin regolith and saline groundwater can cause major densification of subsoils in the class of soil profiles known as duplex soils. Many of these authigenic minerals are deposited within a systematic repetitive pattern within a single landscape or toposequence. The impregnation processes increase the density of regolith and may greatly increase the strength of soil materials (Fig. 13) so that movement of water, air, roots and nutrients are inhibited and diverse land management problems develop in a systematic pattern in the landscape.

SEM and EDS images of a thin section of calcrete/dolocrete from Jerramungup, southwestern Australia. The original highly porous granitic saprolite consisted of a kaolin groundmass with exfoliated kaolin pseudomorphs of mica crystals. The materials have been impregnated by a mixture of calcite and dolomite as is indicated by the X-ray diffraction pattern. Analyses of the original saprolite would have occupied the top apex of the composition triangle but most of the highly porous material has been indurated by 70–100% carbonates that occupy the former porosity. Modified from [6].
Image de microscopie électronique à balayage et de spectrométrie à dispersion d'énergie d'une section fine de calcrète/dolocrète de Jerramungup (Sud-Ouest australien). La saprolite granitique originelle, très poreuse, consiste en un fond matriciel kaolinique avec des pseudomorphes kaoliniques de cristaux de mica exfoliés. Les matériaux ont été imprégnés par un mélange de calcite et de dolomite, comme l'indique le diagramme de diffraction X. Les analyses de la saprolite originelle se placent à la pointe du triangle de composition, mais la plus grande partie du matériau très poreux a été indurée par 70–100% de carbonates qui occupent la porosité antérieure. Modifié d'après [6].
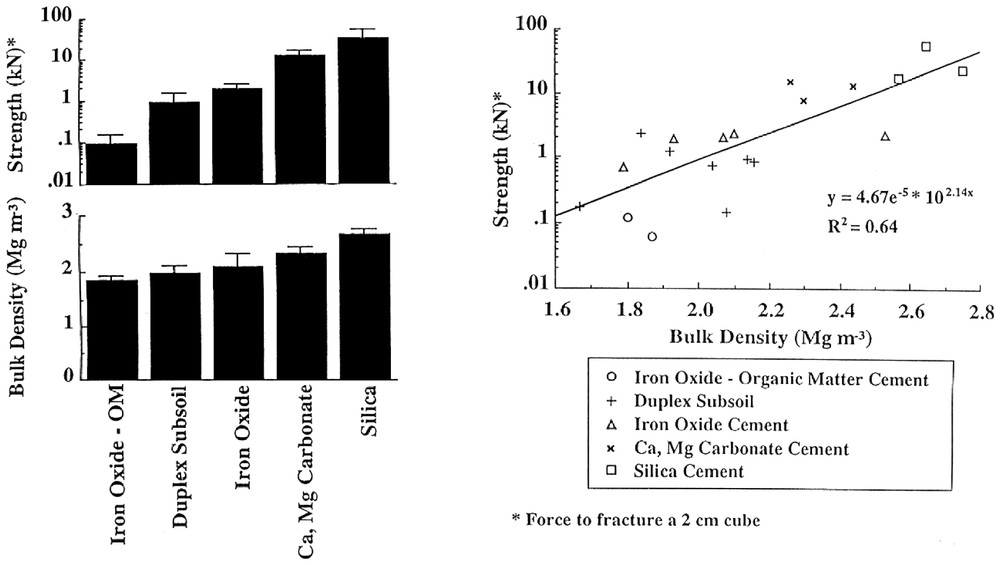
Histograms and bivariate plot showing the bulk density and strength of regolith materials that have been indurated by various cementing materials. Induration produces materials of high density and strength with secondary silicification (silcrete) resulting in extremely high strength values and as the bulk density (about 2.5 mg) values are equal to those of the major silicate minerals that are present, there can be little or no residual porosity.
Histogrammes et coordonnées montrant la densité apparente et la rigidité des matériaux régolithiques qui ont été indurés par une cimentation variée. L'induration produit des matériaux de fortes densité et rigidité, avec silicification secondaire (silcrète), qui est à l'origine des valeurs extrêmement élevées de la rigidité ; comme les densités apparentes (environ 2,5 mg) sont égales à celles des principaux minéraux silicatés présents, il n'y a que peu ou pas de porosité résiduelle.
3 Conclusions
Climate change imposed upon the stable, lateritised low-relief landscape of southwestern Australia has produced a complex assemblage of chemically modified regolith materials. By identifying the processes responsible for generating these materials, we have been able to place them into a spatial and temporal model. The sequence of cross-sectional diagrams in Fig. 14 shows how the stable, vegetated pre-erosion Tertiary laterite surface was eroded as climate became more arid and possibly episodic, and as stabilising vegetation diminished. At this stage erosion and deposition of lateritic materials into colluvial valley side bodies and valley floor sediments were the dominant processes. External drainage still operated to remove dissolved ions so that widespread precipitation of minerals from groundwater had not commenced.




Schematic cross-sections of the landscape at Merredin, southwestern Australia, showing the effects of increasing aridity on lateritic regolith. Modified from [10]. (a) The stable vegetated Tertiary landscape with the dominant regolith process being in situ lateritic weathering of a (seasonally) saturated regolith column and with export of dissolved ions by an active river system. (b) Increasing aridity and possible episodic rainfall lowers the water table and reduces vegetation density leading to erosion of lateritic regolith. Colluvium is deposited on slopes and alluvium on valley floors. The drainage system continues to export dissolved ions. (c) Continuing erosion has removed much of the upper laterite profile and infilled valleys with sediments. External drainage is now greatly reduced. Groundwater silcrete and ferricrete form in low-lying situations and lenses of silcrete in root zones above the water table. (d) During recent arid climate phases, saline groundwater has dominated so that soluble salts occur in valley floor playas (salt lakes) and minerals precipitate in the regolith in a ‘chromatographic’ pattern that reflects the mobility and solubility of ions. Silcrete and ferricrete continue to form together with neoformation of carbonates, sulphates, halite, smectite, sepiolite, palygorskite and many other minerals.
Coupes schématiques transversales de paysage à Merredin, Sud-Ouest australien montrant les effets d'une aridité croissante sur une régolithe latéritique (modifié selon [10]). (a) Paysage tertiaire stable et couvert de végétation, avec le processus dominant de formation d'une régolithe, à savoir altération latéritique in situ d'une colonne de régolithe saturée (de façon saisonnière) et exportation des ions dissous par un système actif de rivière. (b) L'aridité croissante et de possibles précipitations épisodiques abaissent la nappe et réduisent la densité de la végétation, en provoquant l'érosion de la régolithe latéritique. Des colluviums se déposent sur les pentes et des alluvions dans les fonds de vallée. Le système de drainage continue à exporter des ions dissous. (c) La poursuite de l'érosion a évacué la plus grande part de la partie supérieure du profil latéritique et rempli les vallées de sédiments. Le drainage est à présent fortement réduit. Des silcrètes et ferricrètes de nappe se forment dans les zones basses et des lentilles de silcrètes dans les zones racinaires au-dessus de la nappe. (d) Pendant les phases arides récentes, l'eau de nappe saline est prédominante, si bien que les sels solubles s'observent dans les playas de plancher de vallée (lacs salés) et les minéraux précipitent dans la régolithe selon une distribution « chromatographique » qui reflète la mobilité et la solubilité des ions. Les silcrètes et les ferricrètes continuent à se former, ainsi que se développe la néoformation de carbonates, sulfates, halite, smectite, sépiolite, palygorskite et de nombre d'autres minéraux.
With the onset of aridity in the Early Quaternary and/or Miocene/post-Miocene the water table subsided and external drainage became intermittent. At this time lenticular silcrete formed in regolith on slopes where tree extracted water and sheets of groundwater silcrete and ferricrete developed at seepage areas on and near the valley floor. Finally the increasing aridity of the post Miocene [19] with virtually no export of the salts introduced by rainfall and to a lesser extent by weathering lead to the salinisation of some valley floors and most groundwater. This stage of development is associated with formation of silcrete, ferricrete, calcrete, sulphates and other authigenic minerals at specific points in the landscape that reflect the local spatial distribution of hydrological and hydrogeochemical conditions [7]. Indeed, this process resembles a giant chromatograph where a complex solution (groundwater) moves through a porous matrix (regolith) and experiences various chemical (Eh, pH, dissolved ion, evaporation) conditions that have lead to the systematic spatial separation of diverse authigenic minerals. This chromatographic interpretation of landscape geochemical processes has provided the basis for several workers to propose novel approaches to exploration geochemistry [11]. This concept also aids in understanding and managing of the disastrous environmental impacts of rising ground waters in south-western Australia that are due to increased recharge after clearing bush for farming [3].
Acknowledgements
We thank Faron C. Mengler for preparing the diagrams.