Version française abrégée
Les hydrates de gaz sont des substances solides, ressemblant à de la glace, qui existent sous les océans et dans les régions polaires. Ils appartiennent à une classe de grandes molécules, organisées en réseaux complexes, appelées clathrates. Les clathrates forment des cages dans lesquelles peuvent s'insérer des molécules étrangères, stabilisant ainsi la structure hôte. Les hydrates de gaz naturels sont typiquement représentés par des molécules de méthane (CH4) emprisonnées à l'intérieur de cages de clathrate, formées de molécules d'eau (Fig. 1). Des hydrates de gaz ont été répertoriés sous les océans, au large de tous les continents (Fig. 2). Ils ont été également forés, et même échantillonnés, dans les régions froides où le pergélisol persiste d'une année à l'autre, tel au Canada, en Sibérie ou en Alaska. L'intérêt scientifique sur les hydrates de gaz a crû exponentiellement au cours des vingt dernières années (Fig. 3).
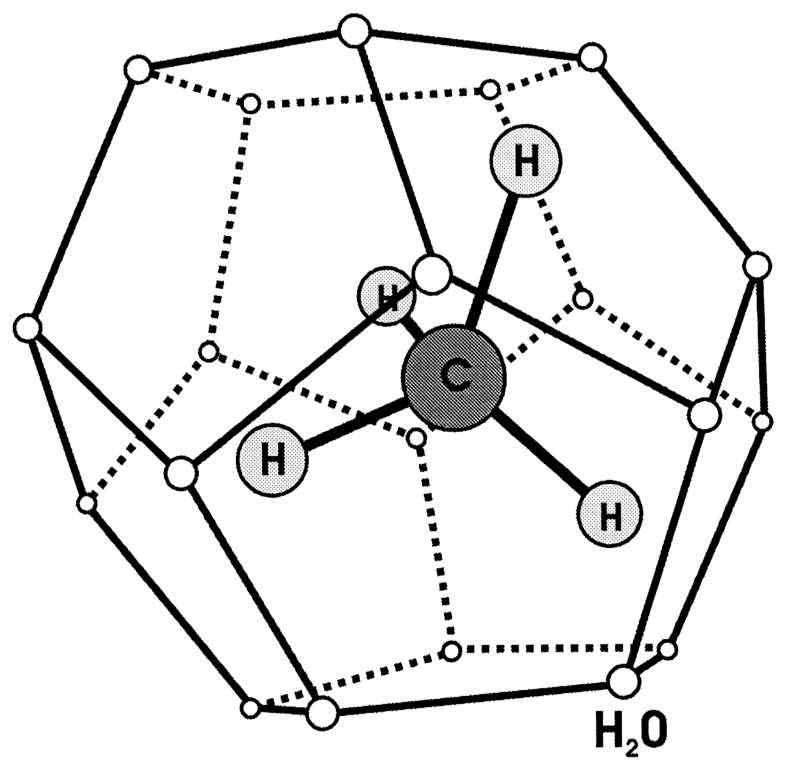
Gas hydrate: methane molecule (CH4) imprisoned within type I clathrate cage formed by water molecules (H2O).
Hydrate de gaz : molécule de méthane (CH4) emprisonnée à l'intérieur d'une cage de clathrate de type I, formée de molécules d'eau (H2O).
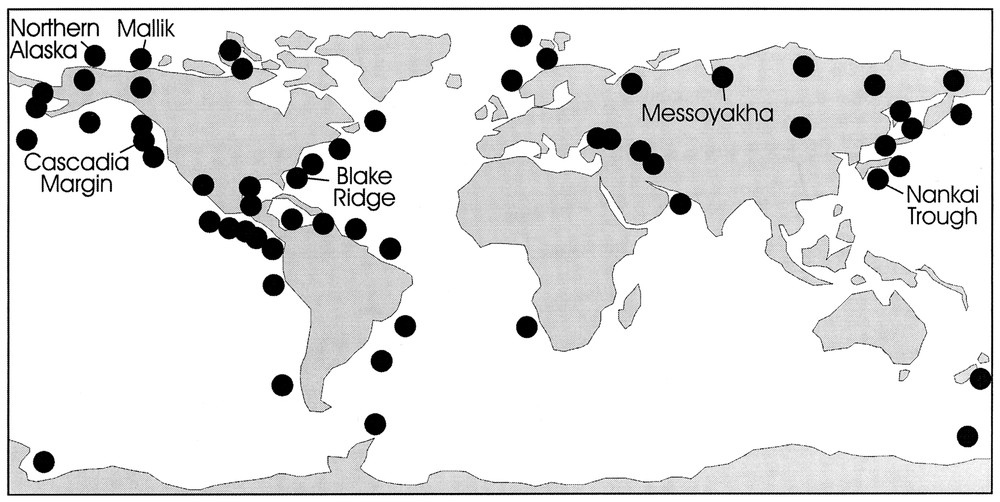
World occurrences of known gas hydrate accumulations. Localities referred to in the text include: Mallik, Blake Ridge, Cascadia Margin, Gulf of Mexico, Nankai Trough et Messoyakha. Modified from various sources.
Distribution mondiale des accumulations connues d'hydrates de gaz naturels. Les localités mentionnées dans le texte incluent : Mallik, Ride de Blake, Marge Cascadia, Golfe du Mexique, Fosse de Nankai et Messoyakha. Modifié à partir de sources diverses.
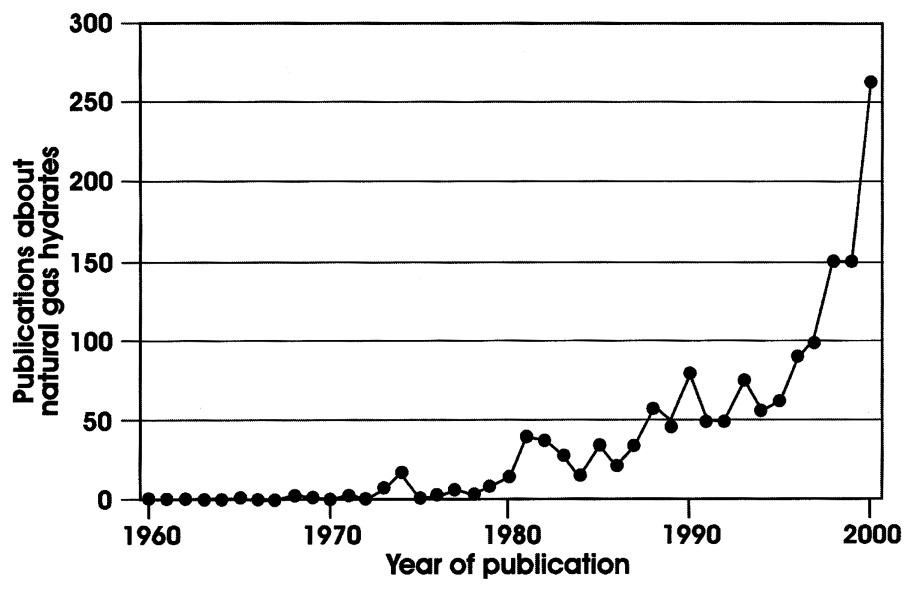
Increase in natural gas hydrate-related publications between 1960 and 2000. Data from American Geological Institute (AGI).
Accroissement du nombre de publications ayant trait aux hydrates de gaz naturels entre 1960 et 2000. Les données proviennent de l'American Geological Institute (AGI).
Les hydrates de gaz se forment en régime de basses températures et hautes pressions. Le champ de stabilité des hydrates de gaz est limitée, d'une part, par la transition entre les phases solides (hydrates) et gazières, et d'autre part, par le gradient hydrothermal dans les océans, et géothermique sous les fonds marins (Fig. 4A). Bien que théoriquement stables à une certaine profondeur dans les océans, les hydrates de gaz ne s'y forment pas en raison de la faible concentration en méthane. Le champ de stabilité, connu sous le nom de Zone de Stabilité des Hydrates de Gaz (ZSHG), s'étend ainsi de la partie supérieure de la colonne sédimentaire jusqu'à quelques centaines de mètres sous les fonds marins. Puisque la concentration en méthane joue un rôle important dans leur formation, les hydrates se concentrent presqu'exclusivement sous les pentes continentales à une profondeur excédant 500 m (Fig. 4A). Sur les continents froids de l'hémisphère nord, la ZSHG gı̂t à des centaines de mètres sous la surface et peut s'étendre à plus d'un kilomètre en profondeur, donc bien en dessous du pergélisol (Fig. 4B). Le champ de stabilité est bordé par l'extrémité méridionale du pergélisol, et au nord, par l'océan Arctique. Dans l'hémisphère sud, tout le continent antarctique est essentiellement sous la ZSHG.
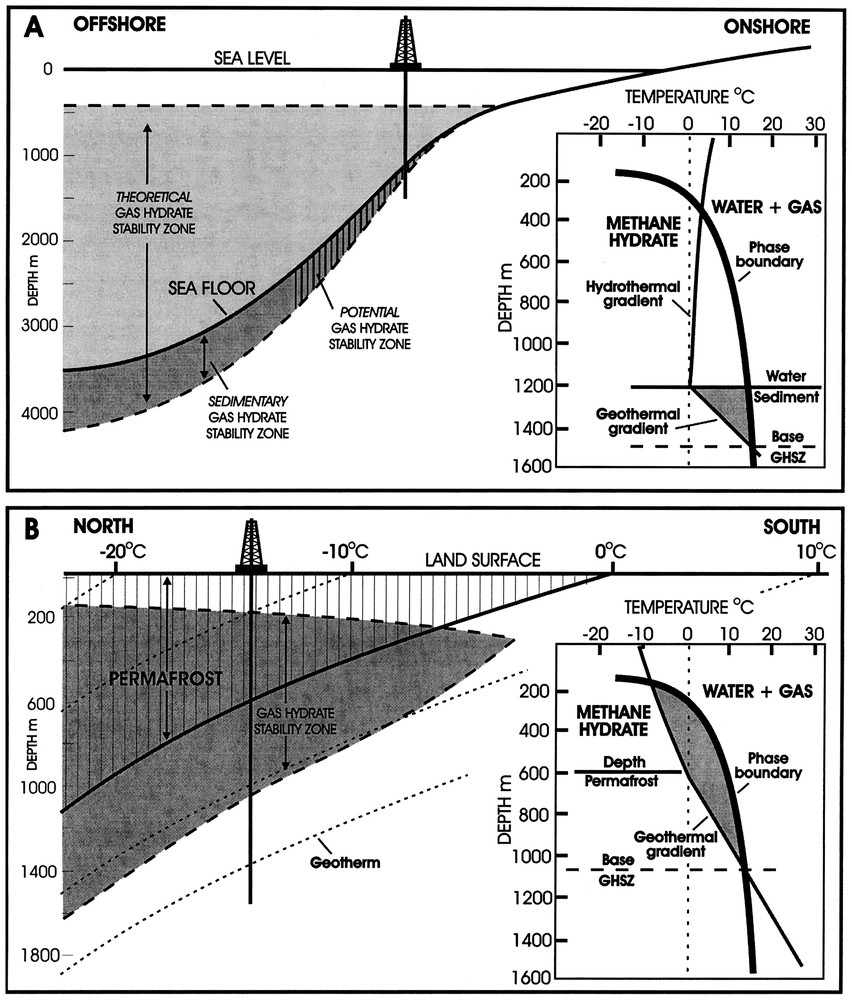
Gas hydrates stability zone (GHSZ), with inserts depicting pressure-temperature profile in wells. Modified from [29]. A. Oceanic GHSZ showing theoretical, sedimentary and potential zones of hydrate formation, B. Continental GHSZ overlapping the permafrost.
Zone de stabilité des hydrates de gaz (ZSHG), avec vignettes montrant les profils de pression et température dans les puits. Modifié à partir de [29]. A. ZSHG océanique montrant les zones théorique, sédimentaire et potentielle de formation des hydrates. B. ZSHG continentale chevauchant le pergélisol.
Les hydrates de gaz se forment au sein de sédiments poreux contenant un minimum de 5 à 10 % de méthane gazeux. Sous cette concentration, la structure de clathrate est instable et ne peut exister bien longtemps. Par contre, une fois formés, les hydrates favorisent la concentration du méthane, et autres gaz, de quatre façons différentes. D'une part, un volume de clathrate séquestre 164 fois son volume en méthane gazeux ; en d'autre termes, 1 cm3 de clathrate emprisonne 164 cm3 de méthane. D'autre part, les sédiments saturés en hydrates forment un barrière d'imperméabilité qui freine, ou tout au moins ralentit, l'échappement de gaz nouvellement formé vers la surface. Ensuite, puisque le méthane a du mal à rejoindre la partie supérieure de la colonne sédimentaire, le lieu de l'oxydation bactérienne, la formation des hydrates ralentit, et dans certains cas empêche, la fermentation microbienne qui normalement détruirait les molécules de méthane. Finalement, avec l'enfouissement, les hydrates finissent par se dissocier à la base de la ZSHG, mais le méthane demeure piégé sous les hydrates.
Trois contextes géologiques différents, et pas nécessairement exclusifs, peuvent engendrer des accumulations importantes d'hydrates. Premièrement, il y a les hydrates qui résultent de la production in situ de méthane, due à la biodégradation de la matière organique dans les premiers 1000 mètres de sédiments. Ensuite, il y a les hydrates qui proviennent d'une « clathratisation » de réservoirs de gaz conventionnels, en réponse, soit à des changements de pression, soit à des changements de température, causés par des évènements tectoniques ou climatiques. Finalement, les hydrates peuvent se former là où il y a des suintements d'hydrocarbures, en milieu tant continental que marin, en interceptant l'échappement gazier vers la surface.
En milieu de pergélisol, les hydrates, une fois formés, seront stables aussi longtemps que la température ambiante restera sous le point de congélation. Par contre, les hydrates formés en milieu océanique sont sensibles, tant aux fluctuations de température, qu'aux changements de pression liés aux fluctuations eustatiques. Un facteur additionnel d'instabilité, particulièrement sous les océans, est la surpression sous la ZSHG qui peut mener à l'expulsion catastrophique des gaz à la surface. De plus, l'instabilité gravitationnelle propre aux pentes continentales, là où se concentrent les hydrates en milieu marin, peut également mener à leur dissociation rapide.
Il existe plusieurs techniques pour identifier la présence d'hydrates de gaz, allant de l'observation directe aux calculs indirects. La méthode la plus largement utilisée consiste à rechercher une réflexion sismique qui imite la surface des fonds marins. Cette surface, connue en anglais sous l'acronyme de BSR pour « Bottom-Simulating Reflector », est due à la présence de gaz libre sous la ZSHG. Le volume d'hydrates au dessus du BSR est quant à lui apparenté au phénomène de brouillage (“blanking”) du signal sismique qui est causé par la présence d'hydrates solides, à vélocité sonique supérieure, dans les sédiments. Des observations directes, sur les fonds marins ou en carottes, ont permis de reconnaı̂tre divers modes de croissance des hydrates. Des techniques de mesures géophysiques, géochimiques et géothermométriques dans les puits permettent de détecter indirectement la présence d'hydrates ou même d'en mesurer la concentration. C'est ainsi qu'on estime qu'il existe plus de carbone séquestré à l'intérieur d'hydrates de gaz (1019 g) que dans l'ensemble de tous les autres réservoirs de carbone, y compris celui représenté par les combustibles fossiles (Fig. 5).
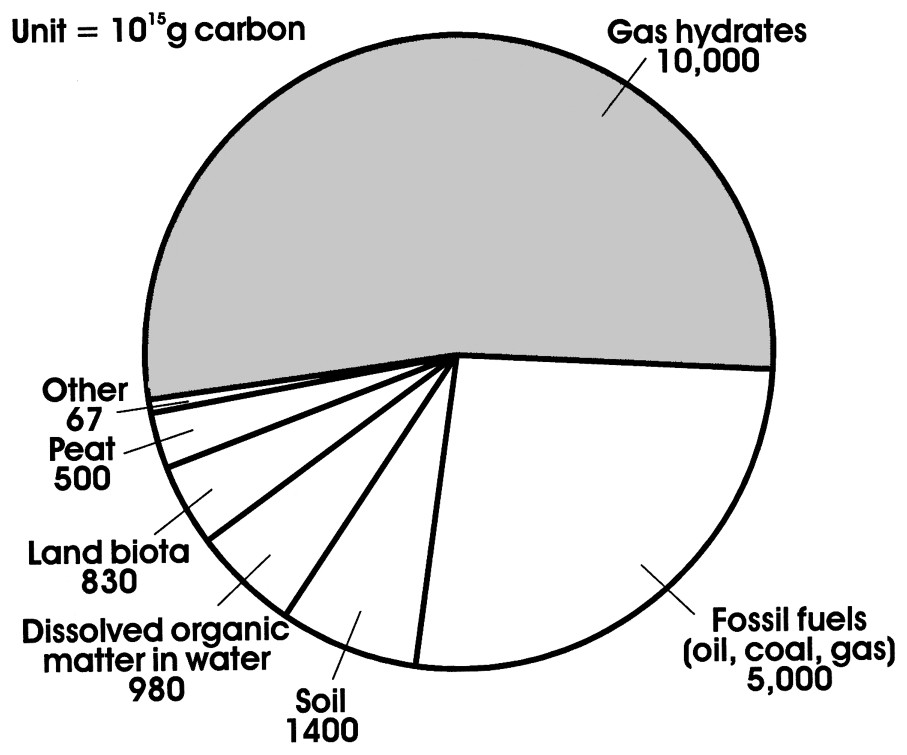
Mass of carbon estimated to be sequestrated as natural gas hydrates compared to other carbon sources. Modified from various sources.
Evaluation de la masse de carbone séquestré sous forme d'hydrates de gaz comparée aux autres sources de carbone. Modifié de sources diverses.
Dans une large mesure, les hydrates jouent le rôle de ciment dans les sédiments non consolidés et donc d'agent stabilisateur. D'aucuns croient que la dissociation des hydrates de gaz, en réponse soit aux changements environnementaux, soit à une future exploitation, pourrait mener à une déstabilisation gravitationnelle, particulièrement le long des pentes continentales, et pourrait donc résulter en des glissements sous-marins fort importants, des tsunamis à répétition, et des dommages côtiers considérables. Il existe, en effet, des évidences que de tels phénomènes ont eu lieu dans un passé relativement récent (Blake Ridge, Etats-Unis ; Storrega Slide, mer de Norvège), et que ceux-ci sont reliés à la présence d'hydrates de gaz. Ce qui est moins certain, c'est jusqu'à quel point les hydrates sont réellement des agents de stabilisation de pente. En fait, le plus grand risque provient probablement plus de la surpression gazière sous la ZSHG, que de l'instabilité et le manque de cohérence associé à l'absence d'hydrates.
Une autre inquiétude reliée aux hydrates de gaz concerne les changements climatiques. En effet, le méthane est un gaz à effet de serre beaucoup plus efficace que le dioxyde de carbone. Le réchauffement global pourrait engendrer la déstabilisation des hydrates dans les océans et en régions de pergélisol, ce qui aurait pour effet le relâchement rapide de quantités énormes de méthane dans l'atmosphère, avec des conséquences catastrophiques pour l'humanité. Le passé géologique offre des exemples probants de réchauffements rapides associés à la déstabilisation des hydrates, le plus étudié d'entres eux ayant eu lieu près de la limite Paléocène-Eocène. Ceci-dit, il n'est pas certain que tous les échappements de méthane provenant des hydrates doivent nécessairement contribuer au réchauffement de l'atmosphère. Selon la rapidité de ce relâchement, il est fort possible, sinon probable, qu'une grande partie du méthane ainsi libéré sera utilisée par des microbes, tant sur les fonds marins, dans les océans que dans les sols, là où le carbone sera éventuellement séquestré. Ainsi, un relâchement de méthane dans l'environnement pourrait tout aussi bien mener à une explosion microbienne, que contribuer au réchauffement global.
Finalement, plusieurs croient que les hydrates de gaz offrent une assurance énergétique pour l'avenir, une conclusion basée sur les énormes volumes de gaz qu'on croit contenus dans les clathrates. Il existe, en effet, des accumulations importantes d'hydrates de gaz sur notre planète, et certaines d'entre elles sont à proximité des populations et des marchés. Par contre, les défis technologiques posés par l'exploitation des hydrates de gaz sont énormes, bien qu'en voie d'une compréhension plus grande. Les techniques d'exploitation consistent à déstabiliser la ZSHG, soit en injectant des inhibiteurs thermodynamiques, tel le méthanol ou le glycol, soit en injectant des fluides chauds pour créer une instabilité thermique, ou encore en relâchant la pression sous la ZSHG en produisant, au départ, le gaz libre et ensuite le gaz résultant de la dissociation des hydrates. Il existe des endroits où la concentration d'hydates est telle, qu'il est permis d'envisager une exploitation commerciale d'ici deux à trois décennies. C'est le cas du champ Mallik dans le Grand Nord canadien [9], là où des expériences ont déjà démontré la faisabilité d'exploitation. Reste maintenant à démontrer la faisabilité économique de tels projets.
1 Introduction
Gas hydrates are icy substances occurring naturally beneath the worlds' oceans and in the polar regions. Because gas hydrates contain vast, and potentially unstable, reserves of methane and other natural gases, they are seen as embracing three of the most important issues in Earth Sciences: energy supply, climate change and natural hazards. However, little is known about the actual resource potential of gas hydrates and the environmental consequences of their, as yet, hypothetical exploitation or anthropogenic disturbance. Thus, the economic and environmental issues that relate to gas hydrates are shrouded in a mythological cloud, embracing both wild resource estimates and doomsday environmental scenarios. The phenomenon of natural gas hydrates does call for more scientific research and real production tests. While the former is ensured through an outburst of activities and ongoing projects around the world, the latter lags far behind, as only one genuine test – Canada's Mallik Project – has been carried out to evaluate the feasibility of exploiting gas hydrates. This paper first reviews the processes of gas hydrate formation in the natural environment. It then addresses the natural or anthropogenic dissociation of gas hydrates and its impact on natural hazards, climate change and energy resources.
2 Formation of natural gas hydrates
2.1 Clathrates and gas hydrates
Clathrates are a class of unusually large, complex networks of molecules forming cage-like structures (Fig. 1). When clathrates are composed of water molecules, they are called hydrates and exist as two fundamental structures (I and II) depending on how many water molecules make up the fundamental building blocks [44]. A hybrid structure (H), combining elements of the two structures also exists [44]. Hydrates are unstable and tend to dissociate rapidly due to the presence of a large empty cavity at the core of their structure. The addition of one or more gas molecules (e.g., carbon dioxide, hydrogen sulfide, etc.) within the cavity, can stabilize the hydrate structure, hence the name gas hydrates. Methane (CH4) is by far the most commonly encountered guest in naturally-occurring clathrates, or natural gas hydrates.
Categorized as oddities during the 19th century and early 20th century, gas hydrates became an engineering concern in the 1930s when it was discovered that they were clogging gas pipelines. It was only thirty years later that naturally-occurring gas hydrates were discovered in gas fields, firstly in Siberia, and subsequently in Alaska [28]. Ever since, gas hydrates have been encountered in a number of deep water boreholes off the coast of the United States, Guatemala, Japan, to name just a few (Fig. 2). They have also been drilled and even cored in the permafrost areas of Canada, Siberia and Alaska. The interest for gas hydrate research has been growing exponentially (Fig. 3), and many countries now harbour a national gas hydrate programme, enticed by their energy potential, or concerned by the potentially-harmful environmental consequences of their exploitation and destabilization.
2.2 Gas Hydrate Stability Zone (GHSZ)
Natural gas hydrates form as solid, icy compounds under a range of low temperatures and high pressures. In the oceanic domain (Fig. 4A), the theoretical area of clathrate stability is delineated by the transition between the solid (hydrates) and gas phases, intersected by the sea water temperature gradient above the sea floor and the geothermal gradient below the sea floor [13]. However, gas hydrates do not form in the ocean due to the low concentration of methane. The sedimentary area of clathrate stability, also know as the Gas Hydrate Stability Zone (GHSZ) is therefore a lens, bounded landward by the point where the field of clathrate stability is no longer intersected by the geothermal gradient. It extends and amplifies oceanward, with increasing water depth, but thins in areas of high geothermal gradients such mid-oceanic ridges or hot spots. However, gas hydrates do not form beyond continental wedges due to sub-critical methane concentrations within the sediments. As a result, the potential area of hydrates formation is a narrow subset of the sedimentary zone of clathrate stability that excludes both sea water and sub-abyssal sediments and lies mostly beneath continental slopes in water depth exceeding 500 m (Fig. 4A). In the permafrost areas of the northern hemisphere (Fig. 4B), the GHSZ, which lies a few hundreds of metres below the surface, is bounded by the southermost extent of the permafrost and to the north by the Arctic Ocean, which, by and large, delineates the northernmost extent of the permafrost, for the exception of a narrow band of subsea permafrost [17]. In the southern hemisphere, all of Antarctica is essentially within the GHSZ.
2.3 Methane concentration
Gas hydrates form in sediment pores with a minimum of 5–10% of gaseous methane [3]. Below that value, clathrates are inherently unstable and dissociate immediately upon formation [49]. Significant gas hydrates concentrations beneath the oceans are often associated with gas seepage, derived from a biogenic or thermogenic gas supply from depths. Gas hydrates are unlikely to form, let alone be preserved, in a sedimentary column with normal methane concentrations. Once formed, however, gas hydrates act as a concentrator of methane and other gases through four different processes:
- – the sequestration of gas molecules by clathrate proceeds through a 164:1 ratio; i.e., 1 cm3 of clathrate captures 164 cm3 of methane [44];
- – sediments saturated with gas hydrates act as a low permeability barrier slowing down gas seeping from depth [52];
- – hydrate formation short-circuits normal fermentation processes as methane no longer reaches the uppermost part of the sedimentary column, let alone sea water, where bacterial oxidation takes place [16];
- – as burial proceeds, hydrates dissociation occurs at the base of the GHSZ freeing gas molecules that remain trapped beneath hydrates [47].
These four processes should theoretically, with time, lead to a fully-saturated GHSZ and an increasingly-rich free gas zone beneath the GHSZ. However, even the most concentrated gas hydrate deposits show pore spaces that are nowhere near full saturation. Only 85% of the porosity available for hydrates at Mallik is actually saturated with gas hydrates, one of the world's richest site [9]. Other known deposits have saturation values that are much less than that of Mallik. Likewise, hydrate-rich zones are not as impermeable as once thought. For instance, free gas migrates upward for some hundreds of metres within the Blake Ridge GHSZ [15]; likewise in the Gulf of Mexico, where microbial communities thrive off free methane bubbling out of hydrate zones [55]. Clearly, some methane does escape onto the sea floor [20], and not just methane released from the surficial dissociation of hydrates. Some of that methane comes from beneath the GHSZ and travels along fractures and faults not quite sealed by hydrates. The release of methane can take place violently, as shown by mud volcanoes and pockmarks [1,46], i.e., crater-like sea floor features associated with brecciated sediments and ejecta. Hence, the GHSZ slows down, but does not prevent, vertical gas migration. As a result, the critical methane concentration necessary for continuing hydrate formation is a balance between methane fluxes at the base of the GHSZ and the capacity of that zone to slow down upward gas migration and ultimate escape to the ocean or the atmosphere.
2.4 Geological conditions
Three fundamental, and not mutually exclusive, geological mechanisms can lead to gas hydrate formation: in situ generation of methane; ‘clathratisation’ of conventional reservoirs; and baffling of gas seepage.
2.4.1 In situ generation of methane
Organic matter-rich sediments can accumulate in marine environments, providing high biological productivity and/or ineffective sea floor oxidation, conditions similar to the accumulation of petroleum source rocks. The biodegradation of such organic-rich sediments could conceivably generate enough methane in the upper 1000 m of the sedimentary column to saturate larger pore spaces within interfingering coarser sediments, and initiate the process of gas hydrate formation, providing the area lies within the GHSZ. Further burial of organic-rich sediments would supply the system with additional methane-generating material and lead to the expansion of an increasingly-impermeable hydrate-rich zone. Ongoing burial would keep feeding the system, while hydrate dissociation of the base of the GHSZ would release free methane at depth.
2.4.2 ‘Clathratisation’ of conventional reservoirs
Conventional gas reservoirs are concentrations of biogenic and/or thermogenic gas trapped and sealed within porous sediments onshore or offshore. More than one geological and/or climatic procesess can bring an existing gas reservoir into the GHSZ. For instance, tectonic uplifts, followed by erosion could take a deep-seated conventional reservoir into the GHSZ [37]. Likewise, burial of a shallow reservoir in a cold permafrost setting would eventually bring it into the GHSZ. Sustained cooling of a conventional gas field below the freezing point could also lead to the ‘clathratisation’ of that field at considerable depths. Shallow submarine reservoirs subjected to increasing pressure, through sea level rise, or decreasing temperature, through sea level cooling, could also lead to an hydrate field. In both cases, however, this process would be essentially restricted to shallow depths where the vertical expansion of the GHSZ with both increasing pressure or decreasing temperature is greatest; at similar shallow depths, a decrease in temperature is far more efficient than an increase in pressure.
2.4.3 Baffling of gas/fluid seepage
Liquid and gaseous hydrocarbons seep to the surface in a variety of marine and continental settings. Seepages are especially active in productive petroleum provinces, such as the Gulf of Mexico or the Caspian Sea [10]. Seepage occurs through diffusion, advection or effusion, depending on a variety of factors, such as porosity, permeability, fracture patterns, fault conduits, lithostatic and hydrostatic pressures, etc. A geological plumbing system that connects a deep-seated oil and gas reservoirs with a higher structural level, or with the surface, can pass through the GHSZ, providing the proper offshore or onshore P–T setting [52]. Gas seepage through the GHSZ by no mean insures gas hydrate formation, but if the seeping gas is trapped, partially or completely, by some porous horizons beneath the surface, the process of hydrate formation can be initiated, and with time, a variably concentrated hydrate zone will develop [48].
2.5 Stability and longevity of gas hydrate systems
Most continental permafrost hydrates will remain stable as long as the temperature is below freezing point. Hence this setting has the potential to sequestrate large volumes of methane as gas hydrates for periods that can easily extend in millions of years, providing a steady supply of gas [31]. Because permafrost gas hydrate deposits lie hundreds of metres below the surface, minor surface temperatures fluctuations are unlikely to disturb gas hydrates, and it will take time to destroy the entire gas hydrate system over continental areas. Given time, large volumes of gas hydrates can form in permafrost settings, yet significant dissociation can also take place due to climatically-sensitive nature of the area of discontinuous permafrost and the large temperature shifts commonly associated with that area. For instance, a temperature increase of 5–10 °C near the limit of discontinuous permafrost can easily translate into hundreds of kilometres of poleward shift for the GHSZ (Fig. 4B).
Oceanic hydrate systems are sensitive to temperature shifts, through climate change, and to pressure change, through sea level fluctuations. The termination of the GHSZ tapering wedge in shallow water is inherently unstable. In contrast, the deeper, more offshore, reaches of the GHSZ are far more stable, as temperatures shifts of more than 1 °C on the deep sea floor are rare, and the pressure changes associated with large eustatic variations are also insignificant. The greatest threat to gas hydrate stability in oceanic settings does not come from minor environmental fluctuations, but rather from the buildup of free gas beneath the GHSZ, which leads to overpressuring and catastrophic release of gas through pockmarks expulsion, volcanoes or surface seepages [22]. Overpressuring does occur beneath the GHSZ in continental settings, but the thick sedimentary column above the hydrate fields confines that pressure to the subsurface. In addition, most oceanic gas hydrates occur along gravitationally unstable continental slopes, adding yet another factor favouring their eventual demise.
2.6 Identification and characterization
Gas hydrates are identified by a variety of techniques, involving direct and indirect calculations, measurements and observations. Calculation of the GHSZ is a first step, and a relatively easy one, providing a knowledge of the geothermal gradient in an area. However, the single most important feature for identifying gas hydrates is the Bottom Simulating Reflector (BSR). The BSR is a sharp impedence contrast on seismic profiles that mimicts the sea floor. The BSR marks the transition between the hydrates zone and the free gas zone beneath it [43,47]. Thus, the absence of a BSR does not necessarily mean the absence of gas hydrates [38], but simply the absence or paucity of free gas. A BSR also does not indicate anything about the volume of hydrates contained above it. This information is indicated by another seismic phenomenon, the ‘blanking’ (or ‘wipeout’) of the signal above the BSR, which is associated with the increased sonic velocity of solid hydrates [42].
Direct observations of hydrate mounds and ridges on the sea floor using submersible, deep towed cameras or side scan sonars have demonstrated the presence of hydrates in many areas. Likewise, gas hydrates have now been recovered in drill cores in several marine and continental areas. Hydrates have been shown to occur as disseminated particles, pore filling cements, zebra-like laminated fabrics, and massive hydrate zones up to 3 m thick. A large variety of downhole geophysical experiments have been attempted to characterize hydrates [23]. Among others, the resistivity and sonic velocities of hydrate zones is characteristically high [5]. Geochemical sampling of water formation has also yielded some clues about hydrate formation and occurrences [51]. For instance, the growth of hydrates does not incorporate chlorine, which results in high salinity readings [53]. Likewise, the growth of hydrates is an endothermic process, which is shown by a temperatures drop in geothermometric profiles [31].
2.7 World occurrences and estimates
It has been estimated that more carbon is contained in gas hydrates than in the totality of all other carbon reservoirs on Earth (Fig. 5), including fossil fuels (but excluding disseminated carbon in rocks and sediments). As much as 1019 g of carbon is believed to be trapped mostly as methane within solid gas hydrates [29]. This astonishing estimate is based largely on the large volume of sediments lying within the GHSZ in the oceans and in permafrost areas, and on extrapolations from a handful of known deposits, which may well be the exception rather than the norm. Much disagreements exist about the veracity of these estimates, and a wide range of figures, departing by several orders of magnitudes from the above estimates, have been proposed [4]. Anyway, the amount of methane contained in gas hydrates is significant, and this very fact has spurred a wide range of interest, and myths, about the environmental, climatic and economic aspects of gas hydrates.
3 Dissociation of natural gas hydrates
Gas hydrates could provide an energy supply assurance for future generations, yet lead to potentially dangerous conditions for the environment, climate and human infrastructures. Each of these aspects, albeit based on genuine observations, is shrouded in clouds of exaggeration. The next paragraphs attempt to decipher between myths and facts, and emphasize the scientific uncertainties around the phenomenon of gas hydrate dissociation, as it relates to natural hazards, climate change and energy supply.
3.1 Natural hazards
3.1.1 Myth
Gas hydrates act as a cement, hence as a stabilizing agent, within unconsolidated, or poorly consolidated sediments [27]. Gas hydrates dissociation, therefore sediment destabilization, coupled with the inherent gravitational instability of the slope sediments that contain them could lead to massive and catastrophic landslides and mass wasting events, with potentially devastating consequences for marine and coastal environments and human infrastructures and populations [18]. Such events could lead to deep sea erosion of significant magnitude as canyons, old and new, would be carved out by sediment gravity flows and turbidites, hence affecting sea floor dwelling biota and commercial fisheries. Likewise, tsunamises associated with such events could lead to massive devastation in the nearby shallow seas and populated coastal areas.
3.1.2 Facts
Experiments have shown that hydrated sediments are stronger than non-hydrated sediments [44], and accordingly, there is evidence that marine slumping has occurred in response to gas hydrate destabilization in the past [39]. More than 200 slump scars can be observed near the termination of the vast hydrate deposits of Blake Ridge, on the eastern seaboard of the United States [15]. These slumps likely occurred in response to destabilization of the GSHZ some thousands of years ago. Similar scars and deposits occur around the world [45], but by far the most impressive is the 7000 year-old Storrega Slide in the Norwegian Sea, Norway, which led to a 290-km long scar, the removal of more than 5500 km3 of sediments and its transportation over 800 km towards the Atlantic Ocean [41]. Since the erosional scar levels out at the base of the GHSZ in the area, it has been suggested that the massive slump, and the transportation that followed, resulted from over-pressured gas at the base of the GHSZ. The gas may have allowed the huge volume of material to glide for several hundreds of kilometers on a low gradient. Evidence of overpressure near the base of the GHSZ is pervasive in the Barents Sea and the North Sea, where thousands of pockmarks have been documented [22]. Luxuriant chemosynthetic communities and methane-derived carbonates occur at the bottom of some pockmarks attesting to the natural gas association [22].
3.1.3 Issues
A priori there appears to be a justification for doomsday environmental scenarios in a handful of modern and recent examples linking gas hydrates to sea floor stability and human infrastructures. Yet the question remains whether humans, especially drilling crews, as well as coastal populations will be put inceasingly at risk [21]. The answer to this comes down as to how much gas hydrates is really contained in the sediments, no matter how extensive the GHSZ is, to what extent gas hydrates act as a cement and a slope stabilizor, and what percentage of gas hydrate must exist in a given sedimentary accumulation to act as such. Many slope areas of the world contain very little or no gas hydrates, yet they appear as stable as could possibly be.
Likewise, whether global warming will make all continental slopes ‘alive’ is open to question, and again it comes down to how much hydrates occur and how stable hydrate-laden sediments really are compared to hydrate-free sediments. Interestingly, the best examples of hydrate-related instability, the Norwegian examples, have very little to do with pressure changes and temperatures shifts associated with environmental changes [21], but much more with the inherent property of the build up of free gas and pressure beneath the GHSZ [27]. That phenomenon probably poses a greater risk to the environment and mankind than destabilization brought about by climate changes. A knowledge of where these overpressured zones exist would go a long way towards reducing the risk in any gas hydrate-related exploitaton. Clearly, the problem of hydrate-related natural hazards should be viewed under the light of risk assessment, just like any other natural catastrophes such as earthquakes or river flooding.
4 Climate change
4.1 Myths
Methane, when released to the atmosphere, is a greenhouse gas much more efficient than carbon dioxide [26]. Global warming will lead to the destabilization of gas hydrates in the oceans and in permafrost areas which will release large volumes of methane in the atmosphere over a relatively short period of time. When compounded with the anthropogenic increase in atmospheric carbon dioxide, the additional greenhouse effect from clathrate methane could send the world climate into a tail spin. Additional factors such as the shutdown of thermohaline circulation, the warming up of the world oceans, and the further catastrophic release of gas hydrates from unstable slope settings [15], would feed into itself (runaway greenhouse) to shift the world climate into an alternate state, marked by very warm and inhospitable conditions on land and sluggish and unproductive world oceans. For many, the idea of exploiting gas hydrates for their ressource potential is akin to playing Russian roulette with the world climate, with dire consequences for humanity [18].
4.1.1 Facts
The rock record does provide a few examples of global warming events likely associated with massive and apparently rapid release of methane from gas hydrates. One of these is the Late Paleocene Thermal Maximum (LPTM), which recorded a large shift in global temperatures over a period of probably less than a few thousand years [11,14]. This event was accompanied by a significant, and geologically rapid, δ13C shift of about 3 per mil in both the organic and inorganic carbon reservoirs simultaneously. Mass balance considerations have suggested a rapid influx of large volume of isotopically-light carbon into the worlds oceans. Methane, the most 13C-depleted carbon compound on Earth (−40 to −50 per mil for thermogenic methane, and as low as −90 per mil for biogenic methane), is the only material that can shift the world ocean's isotopic composition, providing it is released in sufficient amounts. Hence, it is believed that the LTPM was caused, or at least accompanied, by a massive release of gas hydrate methane in the environment. The influx of greenhouse-efficient methane into the atmosphere would have contributed to a positive feedback mechanism, which presumably led to a more than 10 °C increase in many parts of the world as shown by oxygen isotopes of fossils and carbonate sediments. Other isotopic events, often associated with the evidence of global warming and mass extinctions, have been linked to variably large and rapid releases of hydrate methane into the environment. These include some Proterozoic [25], Permo–Triassic [30], Jurassic [18,19,40], Tertiary [27] and Quaternary events [24,26]. It is likely that gas hydrate methane was periodically released throughout the geological past, ranging from the frequent and relatively minor releases on a thousand-year scale, to the rare, yet massive releases every tens or hundreds of millions years.
4.1.2 Issues
Methane seepage on the modern sea floor is always associated with some levels of microbial oxidation [54]. Methane becomes the energy source to a variety of bacterial fauna that can develop rapidly [16], and produce both organic films (e.g., Beggiatoa) and authigenic carbonates that incorporate 13C-depleted carbon [1]. The biogenic oxidation of methane also generates CO2 as a by-product. The CO2 released in the water column either escapes to the atmosphere, and thus contributes to greenhouse warming, or is utilized by the phytoplankton before reaching the surface. Isotopically light carbon dioxide does escape to the atmosphere, but, by and large, much of the methane-derived carbon is transferred to the inorganic and organic reservoirs at the site of seepage. Hence, a synchronous record of 13C-depleted carbonates and organic matter in the sediments does not necessarily indicate a significant release of methane, or even isotopically light carbon dioxide into the atmosphere. A release of methane from gas hydrates is just as likely to generate a microbial bloom in the ocean as global warming in the atmosphere [54].
For methane to be a warming agent it has to by-pass normal fermentation processes, and therefore has to be released very quickly and massively [12]. Neither a slow, progressive release of large volumes of methane, nor a rapid release of small volumes of methane are likely to lead to much global warming, but rather to an outburst of bacterial productivity in the oceans. Accordingly, not all negative C-isotope excursions in the rock record were associated with mass extinctions or even global warming. However, a few natural processes can lead to the rapid dissociation of very large volume of gas hydrates and the equally rapid release of its methane into the oceans and the atmosphere at a rate that surpasses that of bacterial uptake and sedimentary sequestration. Possible mechanisms includes: atmospheric temperature increase, oceanic temperature increase, slope failure [13], methane expulsion [11] and sea level fall. Once in the atmosphere, the residence time of methane is about ten years before it is oxidized and transformed into CO2.
4.2 Energy resources
4.2.1 Myths
The amount of natural gas stored in natural gas hydrates is estimated at about 20 000 trillion m3, a figure nearly two order of magnitudes larger than recoverable conventional gas resources [4,6,29]. Hence, gas hydrates provide an energy supply assurance for the 21st century [32]. Countries that have traditionally relied on oil and gas imports for their energy needs will become self-sufficient because of the vast gas hydrate reserves contained in their nearby continental slopes. Japan will, within the next ten years, commercially exploit gas hydrates from its surrounding offshore basins [35]. Likewise, North Americans need not to worry about energy supply as gas hydrates will fill in the gaps left by conventional exploration. Technology will quickly evolve to make gas hydrate exploitation feasible and economically-viable in a variety of deep water and permafrost settings [34].
4.2.2 Facts
Large volumes of methane are stored as natural gas hydrates on our planet [5]. Significant volumes of methane also occur as free gas beneath most gas hydrate horizons. A variety of techniques have demonstrated the presence of large concentrations of both gas hydrates and associated free gas in proximity to populations and markets. These include Blake Ridge, where a 200-m thick hydrate-bearing zone accumulation is believed to contain as much 1.5 billion m3 of gas per km2, Hydrate Ridge (Cascadia Margin) with 467 million m3 of gas per km2 [50], and Nankai Trough, where as much as 756 million m3 of gas per km2 of gas is believed present. In permafrost areas, Canada's Mallik site contain nearly 5 billion m3 of gas per km2 and similar vast volumes of methane associated with gas hydrates have been estimated in Alaska, Siberia and in the Canadian Arctic Archipelago [32]. Many other areas, both onshore and offshore, are likely to have trapped huge volumes of methane as gas hydrates (Fig. 2): these include: India, Guatemala, China, etc. [33,36].
The technological challenges to destabilize the gas hydrate zone and to extract methane from the subsurface are well understood, and a number of tests, experiments, and numerical models are currently being developed. The techniques involve one or a combination of three procedures:
- – Shifting the thermodynamic phase boundary between solid hydrates and gaseous methane using inhibitors, such as methanol or glycol. Producing methane from gas hydrates using methanol/glycol injections has been attempted in the vast Messoyakha field, with positive results [28].
- – Thermal injection, whereby hot fluids are injected down wells to destabilize the GHSZ and to release the associated methane. It has been recently performed in a genuine, yet short-lived, production test at Mallik in 2002 [8]. The results of this experiment will be released in 2004 [7]. An earlier experiment to shed some light into the hydrate field was conducted in 1998 and the results published a few years later [9]. Numerical models have shown that this technique has some potential under certain conditions.
- – Pressure release, whereby pressure exerted by the free gas beneath the GHSZ is carefully released to destabilize gas hydrates above and free up gaseous methane. Such an experiment was also conducted as part of the Mallik research well [7]. Models have shown that this technique offers the best possibilities for destabilizing the gas hydrate zone at low energetic and environmental costs.
4.2.3 Issues
The huge worldwide estimates of hydrate methane are suspicious at best, and have nothing to do with the likelihood that hydrates will provide energy supply assurance for the future. Far more significant than a worldwide estimate, is a regional estimate, akin to conventional resource assessment [31]. Such assessments are based on the cumulative knowledge of the architecture and petroleum system of a given basin. The gas hydrate system is a subset of the broader petroleum system, and understanding this system is key to understanding whether hydrates have formed, whether they are concentrated enough to be exploited, and whether they are recoverable in any sort of way. A simple knowledge of the vertical and lateral extent of the GHSZ is insufficient, yet many large estimates combine that very knowledge with extrapolations from one or two well-studied sites in a region, hence assuming that these sites are the norm, and not the exception.
Identification of the resource is clearly a challenge. For instance, a well-defined BSR does not guarantee huge reserves. It only means that a free gas–gas hydrate interface has developed. Likewise the presence of one large field in an area does not guarantee the occurrences of others. However, rich conventional petroleum provinces are more likely to be associated with large secondary hydrate fields than hydrocarbon–poor basins. In addition, each accumulation will have its own challenges: there are no ‘conventional’ hydrate accumulations per se. Exploiting gas hydrate concentrations will revolve around the same issues as conventional gas accumulations: What is the porosity and does it hold much gas? Is the permeability high enough to allow dissociated gas to flow to wells? Is the gas hydrate reservoir compartmentalized in a series of pockets or lenses, or does it form one continuous structure? In addition, some problems specific to gas hydrates will surface: for instance, will it be possible to produce methane from very fine, inherently-unstable low permeability sediments in which hydrates provide some form of cohesion and stability? More importantly, will the identified accumulations lend themselves to any of the three extraction/destabilization techniques? Therein, potentially, lies a mountain of difficulties.
Uncertainty about exploitation raises the question as to what percentage of a given gas hydrate reservoir will actually be recoverable. Likewise, what will be the production rate? Will it be comparable to a conventional well, or so slow as to jeopardize the economic feasibility of its very exploitation? These issues speak to the exploitation cost of gas hydrates, which may be small compared to the energetic costs involved. How much would it cost to operate a thermal injection infrastructure in the high Arctic, or in the deep offshore area? What is the energy necessary to exploit methane from gas hydrates, and how does that compare with conventional gas production? Likewise, there is an environmental cost to gas hydrate exploitation, one that is still poorly understood. Inhibitor injection would be very costly, and unlikely to receive approval from environmental review boards; likewise for some of the large thermal injection facilities necessary for commercial exploitation. By any standard, exploiting gas hydrates will not come cheap, economically, energetically or environmentally.
Equally important are economic considerations, such as market proximity, political stability, terrorism threats, and competing energy resources [2]. Just like conventional gas, not all gas hydrate deposits, no matter how large they are, are close to markets, to petroleum infrastructures, to pipelines, etc. Gas hydrates fall in the category of non-conventional gas resources, and as such, will have to compete with a variety of other non-conventional hydrocarbon resources in the 21st Century. These include deep basin, tight and basin center gas, deep offshore, subsalt plays, coal-bed methane, tar sands, heavy oil, etc. This list also includes other forms of energy, including nuclear which might become more attractive. The 21st century will see the rapid decline of inexpensive oil and gas, and different countries will have to adapt to the reality of far more expensive energy from a variety of sources. What role gas hydrates will have to play remains to be demonstrated. While the resource undoubtedly exists, only time will dictate whether technology, money and markets combine to make hydrates the energy of choice. However, as coal-bed methane has demonstrated through the technological challenges it had to overcome, it might be possible one day to exploit ‘cold-bed’ methane hydrates for the resources they contains.
5 Conclusions
Beyond the wild considerations, doomsday environmental scenarios and overly optimistic resource estimates, gas hydrates are more than a curiosity. They do have a bearing on environmental issues and they may represent a potential supplement to conventional gas production. One cannot escape the fact that they represent literally frozen, yet transient, accumulations of methane in the natural environments, some of which are in proximity to populations, infrastructures and markets. To say that gas hydrates require more research goes without saying, and this is indeed happening. Likewise, real production tests must take place before too much can be said about the resource potential of hydrates. Scientific research and production tests will hopefully take gas hydrates away from the mythical cloud that enshrouds them, to something closer to a well-understood reality. That being said, no one should shy away from these enigmatic substances and downplay the potential quantum leap that may be achieved through technological innovations.
Acknowledgements
This contribution is based upon a presentation by the author before the Scientific Board of the French Petroleum Institute in Paris, November 2002. The author wishes to thank Madame Jacqueline LeCourtier et Messieurs Claude Mandil, Jean Dercourt and Bernard Tissot for their encouragement.