1 Introduction
1.1 The Cambrian Explosion
Early Cambrian time, punctuated by a unique and dramatic series of geological and biological events, has fascinated and puzzled geologists and paleontologists for more than a century [109,110]. Widespread preservation of a detailed animal fossil record was facilitated by the almost simultaneous evolution of the ability to precipitate biominerals such as calcium phosphates and carbonates in nearly forty phyletic-level groups of animals [6,58,71]. While this abrupt appearance of diverse bodyplans in the fossil record was once thought to be coincident with the radiation of animal phyla, recognition of the Late Precambrian, soft-bodied Ediacaran fauna first suggested a divergence of various lineages deeper in geologic time [38].
Today this divergence preceding radiation is further supported by the presence of putative Mesoproterozoic animal trace fossils [35,89,98,118]; however, the apparent lack of a continuous fossil record for these traces suggests that they did not survive for long, possibly experiencing population bottleneck or going extinct during global catastrophes such as “Snowball Earth” events. Molecular-clock analyses have not yet converged on a consistent and robust divergence date: estimates for the main divergence of lineages range from Paleoproterozoic to Vendian time [4,7,12,13,23,30,37,45,72,88,94,101,112,113].
Furthermore, as indicated in Fig. 1, a nearly 50-year investigation of the Ediacaran fauna has revealed only a low diversity of forms [82]. There is even some concern that many of these animals might not be related to extant phyla [96,97]. Either way, the low diversity of Ediacaran fauna stands in stark contrast to the high morphological diversity displayed by Early Cambrian animals. Considering further the unexpected low diversity of forms preserved in otherwise appropriate and promising Neoproterozoic taphonomic settings [114–117], it seems likely that major changes in morphology and biochemistry actually took place during the Cambrian period. Hence, a major component of the massive diversification of life previously termed the ‘Cambrian Explosion’ does belong indeed to the Cambrian!
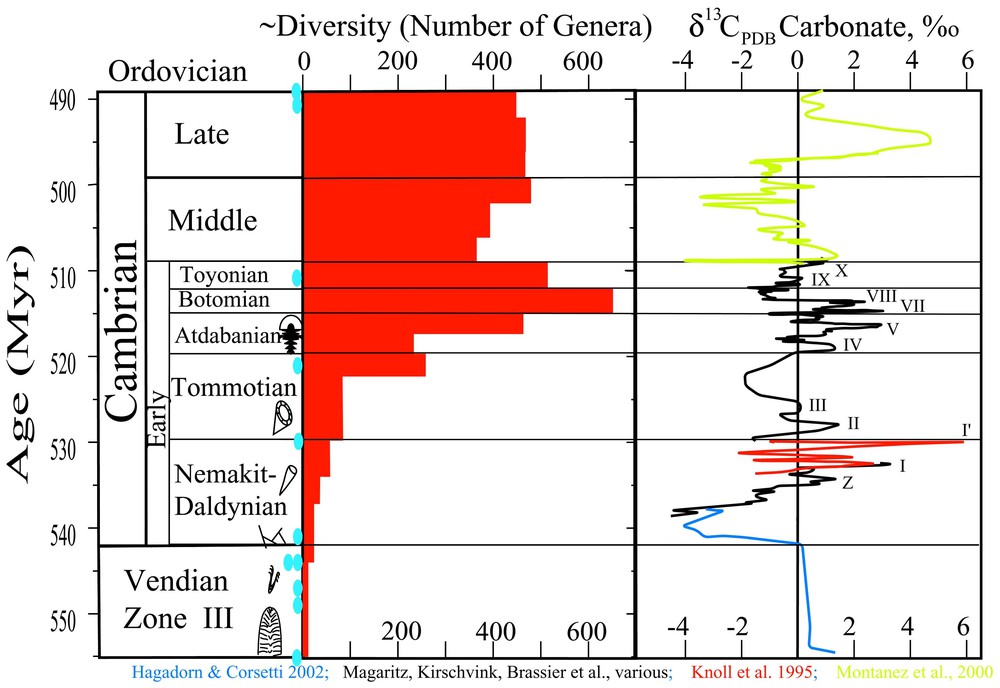
Age constraints, biological diversity, and variations in inorganic carbon isotope ratios for Late Vendian and Cambrian time. Blue ovals along the left indicate the stratigraphic location of volcanic ashes that have been dated using U/Pb on zircon. Carbon isotope values from carbonate were compiled as follows: Blue curve from Corsetti and Hagadorn [18,19,42]; Black are the original data from Siberia [9,11,75,76]; red is the sub-Tommotian gap filled by Knoll et al. [65]; and Green is from the compilation of Montanez et al. [79]. Masquer
Age constraints, biological diversity, and variations in inorganic carbon isotope ratios for Late Vendian and Cambrian time. Blue ovals along the left indicate the stratigraphic location of volcanic ashes that have been dated using U/Pb on zircon. Carbon isotope values ... Lire la suite
Contraintes d'âge, diversité biologique et variations dans les rapports isotopiques du carbone inorganique pour la période Vendien récent–Cambrien. Les ovales bleus sur la gauche indiquent la localisation stratigraphique des cendres volcaniques qui ont été datées par la méthode U/Pb sur zircon. Les valeurs isotopiques du carbone du carbonate ont été compilées comme suit : bleu, données de Corsetti et de Hagadorn [18,19,42] ; noir, données originales de Sibérie [9,11,75,76] ; rouge, lacune sous-tommotienne, comblée par Knoll et al. [65] ; vert, la compilation des données de Montanez et al. [79]. Masquer
Contraintes d'âge, diversité biologique et variations dans les rapports isotopiques du carbone inorganique pour la période Vendien récent–Cambrien. Les ovales bleus sur la gauche indiquent la localisation stratigraphique des cendres volcaniques qui ont été datées par la méthode U/Pb sur ... Lire la suite
1.2 Geochronology
An age estimate of ∼610 million years ago (Ma) for the first appearance of trilobites [16] once implied that the Cambrian radiation event might span an interval of nearly 100 million years. However, an interbedded, zircon-bearing volcanic ash in the pre-trilobite sediments of Morocco (collected by JLK in 1988) yielded a U/Pb age of less than 525 Ma ([17], but see earlier hints for a shorter Cambrian duration [84]). Numerous U/Pb dates from stratigraphically bound zircon-bearing ash units now confirm that only ∼30 million years separate the first appearance of trilobites and the first appearance of graptolites as indicated in Fig. 1 [8,17,26,41,49,69,70]. Concern that the continued contraction of Cambrian time might lead it to vanish entirely prompted the IUGS/IGCP working group on the Precambrian–Cambrian boundary to define the Cambrian Series base over 6 km stratigraphically below the first trilobites, at the present GSSP in Newfoundland [21]. This revised position, marked by the first appearance of a vertically-burrowing trace fossil, Treptichnus pedum, is now dated, in Namibia, at ∼543 Ma [41].
Compared to the duration of evolutionary events later in Earth history, this ‘shrinking’ of the Cambrian geologic timescale indicates an intrinsic background rate of speciation approximately twenty times higher than the average speciation rate for all Mesozoic time – well in excess of the ∼five-fold increase estimated by earlier studies [31]. Such recognition, based on recent, new constraints on the geologic timescale, demands re-examination and evaluation of possible relationships between several geologically unique events that occurred coincident with the Cambrian Explosion.
1.3 Cambrian carbon cycles
As compiled here in Fig. 1, one of the most puzzling and as yet unexplained features of the Cambrian Explosion is the sequence of over a dozen accompanying oscillations in inorganic δ13C values preserved in carbonate, with typical negative-shift magnitudes of
No other set of multiple, high-frequency carbon-isotope perturbations of comparable magnitude, frequency, and duration (∼15 to 20 million years) has been documented in all Earth history. The dominant cause of most of these oscillations is as yet unknown; they represent one of the major puzzles associated with the Cambrian explosion.
We must emphasize here that the Cambrian carbon cycles are robust features of the geological record. After their discovery in the mid 1980s [75,107], they subsequently correlated stratigraphic sequences between various Early Cambrian sections worldwide, thereby solving a long-standing problem of faunal provinciality that had inhibited selection of a Precambrian/Cambrian boundary stratotype [21]. Fig. 2 shows a typical example of this correlation ability for three sections of Tommotian and Atdabanian age on the Siberian platform, demonstrating the robust ability of these carbon cycles to provide detailed stratigraphic correlation.
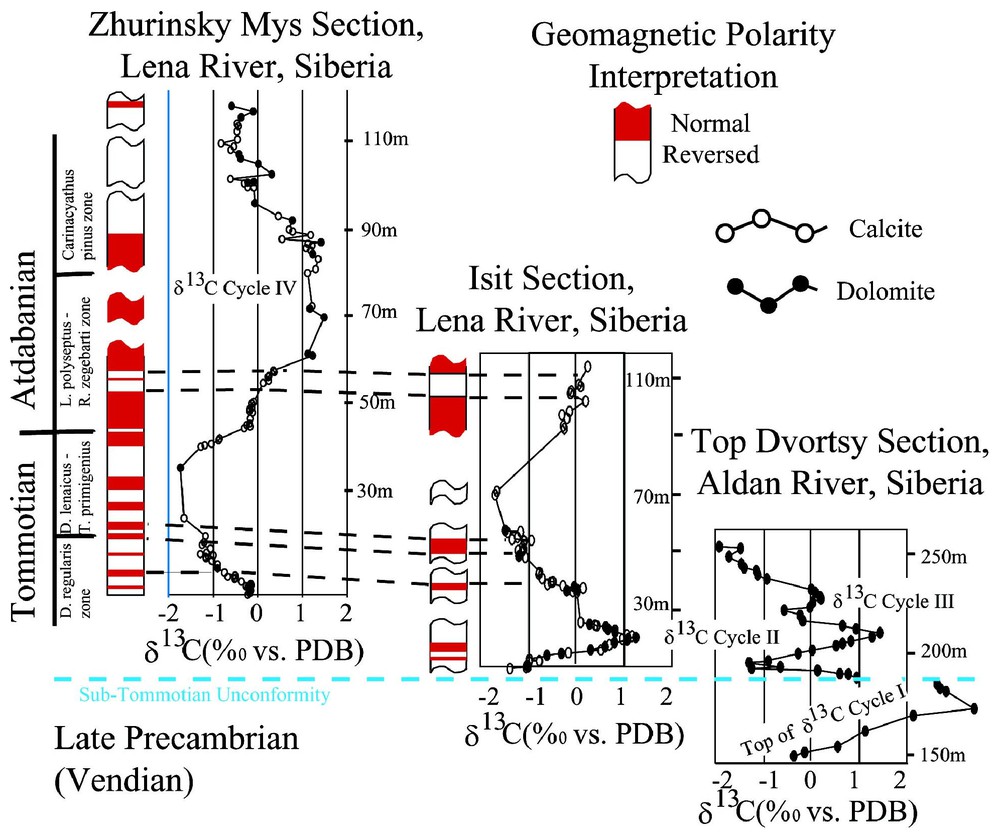
Example of stratigraphic correlation using combinations of carbon isotope, paleontology, and magnetostratigraphy during Early Cambrian time. Note that the tie-in between the carbon data and magnetostratigraphy is exact, because selected fine-grained end-chips from the paleomagnetic samples of Kirschvink and Rozanov [60] were used by Magaritz et al. [75] and Kirschvink et al. [61] as the discovery samples which define the first five Cambrian Carbon Cycles. Figure is updated from [61].
Exemple de corrélation stratigraphique utilisant des combinaisons entre rapports isotopiques du carbone, données paléontologiques et magnétostratigraphiques pendant le Cambrien ancien. À noter que la liaison entre les données relatives au carbone et magnétostratigraphiques est exacte, car des termes à grain fin, sélectionnés dans les échantillons paléomagnétiques de Kirschvink et Rozanov [62] ont été utilisés par Magaritz et al. [75] et Kirschvink et al. [61] en tant qu'échantillons de recherche définissant les cinq premiers cycles du carbone du Cambrien. Figure d'après [61], mise à jour. Masquer
Exemple de corrélation stratigraphique utilisant des combinaisons entre rapports isotopiques du carbone, données paléontologiques et magnétostratigraphiques pendant le Cambrien ancien. À noter que la liaison entre les données relatives au carbone et magnétostratigraphiques est exacte, car des termes à grain fin, ... Lire la suite
Where local or regional paleo-environmental factors render absolute isotopic values different in magnetostratigraphically defined but spatially separated, synchronous sections, the position and sense of isotopic swings, maxima, and minima remain constant. Coexisting organic carbon (kerogen) records show expected fractionation with respect to inorganic carbon-isotope values [36,64,103,104]; where kerogen is absent, implied C-isotope correlation schemes are supported by other, independent tests (for a discussion, see [100]).
The unique style and pattern of these carbon oscillations, their detailed agreement with both paleontological and magnetostratigraphic constraints across nearly 400 km on the Siberian platform, and their invariance with dolomite/calcite ratios and other lithological factors argue persuasively that they record a primary signal of the Cambrian oceans [61]. When viewed in detail, individual Cambrian carbon cycles do bear some relation to biological evolution. For example, Hagadorn and Corsetti [19,42] note that the first sharp drop at the basal Cambrian (Nemakit–Daldynian stage) is followed closely by the initial evolution of vertical burrowing behavior. The subsequent global rise in δ13C values is associated with the progressive radiation of molluscan-type fauna [65]. Similarly, sharp drops in δ13C following carbon cycles II, III, and IV are followed by radiation of the archeocyathids, arthropods, and echinoderms, respectively, during Tommotian and Atdabanian time [61]. The sharp drop at the end of the Toyonian [79] is associated with the extinction of the archeocyathid fauna.
Historically, two general hypotheses propose biological association with these carbon cycles. In one, carbon cycles are driven by expansion and contraction of the biosphere itself [74,76]. In the other, carbon shifts directly fingerprint major climatic and geologic (physical) events on Earth's surface [79]; the biosphere presumably responds in accordance with observation, though specific selection pressures are not explicated [62].
1.4 Paleomagnetic data and true polar wander
Many investigators note that Cambrian time appears to be characterized by high dispersion among paleomagnetic pole positions determined for most major continental blocks [55,56,61,63,106,108]. Prior to 1997, few, if any, new age and stratigraphic-correlation constraints were integrated into the existing paleomagnetic database. Attempts to resolve the Cambrian dispersion problem by using numerical smoothing techniques yielded Apparent Polar Wander (APW) paths in disagreement with both geological observations and the best-constrained subsets of the data itself (see the discussion in Kirschvink et al. [62] and analysis in Evans et al. [33] comparing the uncorrected, temporally and spatially-smeared database for the Gondwanaland continents with that corrected for recent age and tectonic constraints).
Such application of updated age constraints reveals intrinsic rates of Cambrian apparent polar wander exceeding those expected from normal plate tectonics. A major part of these plate motions occurs during the time interval encompassing major Early Cambrian biological diversification [62].
Contentious suggestions that the Siberian APW path does not show such Cambrian motion [105] seemed to belie both that paleomagnetic data and its possible import for evolution. Ultimately, erroneous and arbitrary classification criteria used in the former Soviet database [59] obscured recognition of rapid Siberian APW. Furthermore, Early Ordovician tectonic deformation on the Siberian platformal margin completely or partially overprinted characteristic magnetic components, challenging simple determination of primary directions. Subsequent reinvestigations using experimental and statistical approaches designed to resolve such superimposed components demonstrate that large Early Cambrian APW motions comparable to those found on all other continents [54,87] do indeed exist in Siberia, confirming earlier investigation [61]. Kirschvink et al. [62] suggest that this burst of Early Cambrian, apparently rapid plate motion is reasonably explained by an episode of True Polar Wander resulting from an interchange event in Earth's moment of inertia tensor (termed an Inertial Interchange True Polar Wander, or ‘IITPW’, event).
All planets must spin about their principal moment of inertia [39,40]. Since inertial axes are determined by geodynamic processes on and within a planet, over time the magnitude of the principal moment of inertia (the largest eigenvector of the inertial tensor, defining one of three mutually perpendicular inertial axes) may weaken and eventually become equal to the intermediate moment of inertia, producing instability. Relative to its spin axis, the solid part (the mantle and crust) of such a planet can decouple from the remainder (the core) of the planet at a viscosity discontinuity (such as D″) and move relatively freely around the axis of the minimum eigenvector, which is always located on the equator. Thus relatively small changes in inertial configuration can lead to large shifts in the entire solid Earth relative to its geomagnetic field (produced in the liquid outer core). Angular jumps may reach 90° over time intervals as short as 5–15 million years [2,62,90].
IITPW events also predict consequent, non-eustatic sea-level fluctuations that agree to first-order with the geological record of Cambrian relative sea level changes [80]. Cross-continent comparison in an IITPW reference frame furthermore deduces an absolute paleogeography (both paleolatitudes and paleolongitudes constrained by paleomagnetic data to within ∼103 km [62]). Such calculated paleogeography comfortingly yields a close approach between North America and the South American margin of Gondwanaland in Early Paleozoic time, supporting earlier suggestions [24,25].
Evans [32] notes that when Earth's principle and intermediate inertial moments are nearly equal, relatively small geological perturbations can induce IITPW events. In such a case, a single, isolated IITPW event is not any less conceivable than a series of multiple events in which the Earth pivots to and from about the minimum inertial axis. Intriguingly, the locus of Vendian and Cambrian paleomagnetic poles for North America (which, of all continents, displays the best-resolved APW dataset) describes an untrended, great-circle swath: time-sequential poles alternate between low and high paleolatitude. Such a pattern, if founded on reliable and well-time-resolved paleomagnetic data, is expected for multiple IITPW events but as yet unexplained by other scenarios. As detailed below, such motion of Laurentia from equatorial latitudes during Cryogenian glaciations [57] to a position near the South Pole during Mid-Vendian time, and back to the Equator during the Early Cambrian provides a mechanism for creating, storing, and ‘exploding’ methane ‘time bombs’ capable of producing the observed carbon cycles.
2 Methane-clathrate release and the Cambrian: A testable hypothesis
2.1 The Late-Paleocene thermal maximum (LPTM)
The LPTM event coincides with a sudden negative spike in inorganic δ13C of magnitude comparable to that of the smaller Cambrian carbon cycles. Many exquisitely preserved deep-sea sediment cores span this interval and record both shallow-water and deep-sea carbon- and oxygen-isotope profiles. Zachos et al. [119] provide an excellent review of the globally-averaged stable isotope trends, rhythms, and aberrations from the Cretaceous–Tertiary mass extinction event 65 million years ago to the present – a time interval comparable in length to that of the Late Vendian through Cambrian interval.
Characteristically, the LPTM event records a sudden drop in bulk carbonate δ13C by
Biogenic methane is produced by a group of Archaea (the methanogenic Archaeobacteria) during anaerobic metabolism of organic compounds; this biochemistry gives it isotopically light δ13C values of ∼−60 to −80‰. Hence, quantifiable perturbation of the oceanic carbon-isotope balance requires volumetrically smaller amounts of organic carbon in methane than in other phases. Such perturbation does not necessarily require massive changes in net oceanic productivity or in biomass.
Methane hydrate at 0 °C is stable in the oceans at depths below ∼300 m; with increasing pressure, this stability temperature rises to ∼24 °C at 4 km [29,67]. Methane clathrates are found on most continental shelves where bottom waters are chilly and where methanogens are active in underlying sediment. Methane hydrate remains stable in these sedimentary reservoirs for long periods of time, but, with increasing burial depth, heating from the geothermal gradient will eventually move deeper layers out of the clathrate stability field (at ∼2 km water depth, the critical sediment burial is ∼500 m).
Until an entire clathrate field passes through burial inversion to free gas, a dynamically unstable situation persists, in which deeper sediments are over-pressurized with gas, but are covered by hundreds of meters of clathrate-cemented sediment. Small seismic events or other physical disruption such as erosion can lead to sudden and dramatic sediment failure, releasing large volumes of methane gas. Such events may even be responsible for generation of destructive tsunamis [68].
Estimates from carbon-cycle models suggest that ∼1018 g of methane are needed to produce the observed LPTM isotopic signal in a modern-ocean model [28], a quantity we approximate by a clathrate ‘ice cube’ ∼20 km on edge. Although this quantity represents about 8% of conservative modern estimates of methane clathrate reserves, it is only a small fraction of the global carbon budget (including carbon locked in sediments and rocks; e.g., [67]).
Data from seismic stratigraphic studies provide strong support for this hypothesis: extensive volumes of Latest Paleocene sediment have been identified as disrupted by methane release. ODP drillcore at site 1051 on the Blake Nose penetrates the seismically disturbed interval; a disrupted mud-clasts lithology is intimately associated with the LPTM event [50,51].
2.2 Cambrian comparison
Carbon isotope variations during the Tertiary are much smaller in amplitude and fewer in number than analogous variations during the Neoproterozoic–Cambrian interval. The largest long-wavelength Tertiary oscillation (from Mid-Paleocene through Early Eocene) has an amplitude of only about 2.5‰ [119], whereas some of the Cambrian oscillations (e.g., I′) have swings of up to 8 to 10‰. In terms of shorter, faster excursions, the LPTM event is within a factor of two or so of the duration of some of the highest-frequency Cambrian cycles (e.g., II, III, V through X).
Hence, if deposits of methane clathrate are responsible for the Cambrian carbon cycles, several conditions must be satisfied: (1) Vendian–Cambrian time must support larger source areas for methane formation than the Cenozoic; (2) a plausible geological situation should lead to the conversion and accumulation of methane as gas hydrates; and (3) some global process must facilitate the episodic release of these overpressurized reservoirs over a long interval of time (randomly ‘detonating’ the methane ‘bombs’).
2.2.1 Evidence suggesting massive production of methane
Neoproterozoic carbon-isotope values from carbonates are unusually positive, with typical pre-glacial and inter-glacial values of about +5 to
We suppose that, as today, significant fractions of such organic carbon would be converted to methane by methanogenic Archaea; at appropriate depths and temperatures this gas would be trapped in the form of methane hydrate. Further assuming basic similarity to the Tertiary carbon cycle conditions, since no Tertiary carbon-isotope highstand approaches such heavy values, the Neoproterozoic and Cambrian organic carbon record attests to reservoirs much larger than those present in the Tertiary or on modern Earth.
2.2.2 Accumulation space for methane hydrates
Efficient accumulation of methane hydrate requires burial of organic-rich sediments and bacteriogenic-gas diffusion upward into an area within the clathrate stability field. Organic sediments typically accumulate on shallow tropical shelves which are neither deep nor cold enough to stabilize clathrate; thus conditions today for clathrate accumulation, such as in the modern-day Caribbean, are relatively rare (today, significant Arctic-shelf clathrate reservoirs likely exist but lie inaccessible and undiscovered). Neoproterozoic ocean basins, however, may have been more conducive to clathrate stabilization.
Schrag et al. [95] note that the post ∼900 Ma presence of widespread passive margins at low latitude following the Neoproterozoic breakup of the supercontinent Rodinia and the equatorward drift of its fragments [34,57] may suggest enhanced susceptibility to local- or regional-scale anoxia. In this scheme, enhanced weathering of tropical landmasses increases riverine carbon and phosphorus inputs into nutrient-rich waters. Efficient recycling of phosphorus in anoxic basins allows for enhanced productivity and greater relative burial of organic to inorganic carbon [95].
Since, by Latest Vendian time, large areas of these organic-rich Laurentian and West Gondwanan rifted margins had moved (we suggest via true polar wander) from the tropics to high southerly latitudes, their methane-clathrate holding capacity was likely optimized (Figs. 3 and 4). During the ∼50 million years separating the termination of the Marinoan glaciation and the base of the Cambrian, large reservoirs of methane hydrate might expectedly accumulate in equilibrium with polar conditions.

IITPW reconstructions for Early and Mid/Late Cambrian time, adapted from Kirschvink et al. [62]. The areas located initially at high latitudes (above 60°) are shaded blue on both diagrams. The large ⊕ marks in Antarctica and Siberia are the approximate locations of the axis of the minimum moment of inertia, around which the solid part of the planet spins during the TPW event.
Reconstitutions d'IITPW pour le Cambrien ancien, moyen et récent à partir des travaux de Kirschvink et al. [62]. Les secteurs situés initialement à des latitudes élevées (au-dessus de 60°) sont ombrés en bleu sur les deux diagrammes. Les grands signes ⊕ sur l'Antarctique et la Sibérie correspondent aux localisations approximatives du moment minimum d'inertie, autour duquel tourne la partie solide de la planète pendant l'événement TPW.
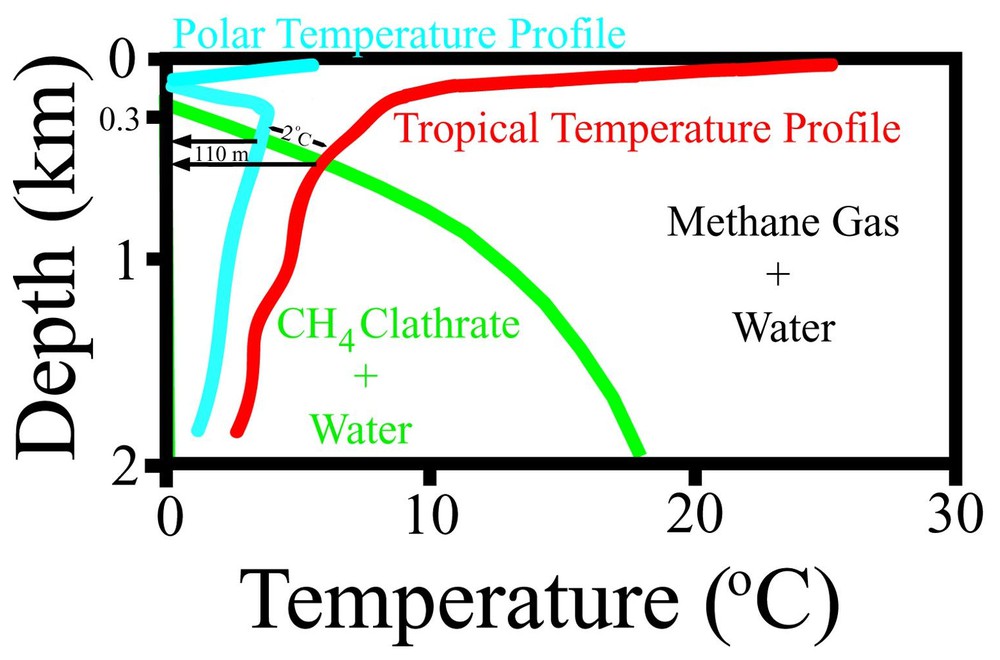
Conservative, schematic tropical and polar water temperature profiles superimposed on the methane clathrate stability field. Note that in modest shelf-and-slope facies, the clathrate-free gas phase boundary shows relative sensitivity to changes in pressure over changes in temperature. An IITPW-induced non-eustatic sea-level rise of ∼110 m during Cambrian pole-to-equator transit (see [80]) would destabilize clathrates because of even moderately higher water temperature, depending on depth. Figure is modified from http://woodshole.er.usgs.gov/project-pages/hydrates/what.html and from http://www.msc.ucla.edu/oceanglobe/pdf/thermo_plot_lab.pdf.
Profils schématiques conservatifs de température de l'eau tropicale et polaire, superposés au champ de stabilité du clathrate de méthane. À noter que, dans les faciès de talus et de pente modestes, la limite de la phase dépourvue de clathrate est relativement plus sensible aux variations de pression que de température. Une élévation non eustatique d'environ 110 m du niveau de la mer, induite par l'IITPW, pendant le transit pôle–équateur du Cambrien (voir [80]), déstabiliserait les clathrates, en raison d'une augmentation, même modérée, de la température de l'eau, en relation avec la profondeur. Figure d'après http://woodshole.er.usgs.gov/project-pages/hydrates/what.html et http://www.msc.ucla.edu/oceanglobe/pdf/thermo_plot_lab.pdf, modifiée. Masquer
Profils schématiques conservatifs de température de l'eau tropicale et polaire, superposés au champ de stabilité du clathrate de méthane. À noter que, dans les faciès de talus et de pente modestes, la limite de la phase dépourvue de clathrate est ... Lire la suite
2.2.3 Sedimentary burial and overpressurization
At typical sedimentation rates along passive continental margins of approximately ten meters per million years, we expect ∼500 m of sediment to accumulate in a 50-million-year interval. At this depth, the geothermal gradient brings a sedimentary package out of its clathrate stability field. Notably, at the start of the Cambrian IITPW event large areas of Laurentia and Gondwanaland would be subjected to additional sedimentation as they slid from the pole toward the equator and into the carbonate belt. These areas would also experience an IITPW-induced non-eustatic sea level rise [62].
2.2.4 Episodic release
The epistatic nature of global circulation patterns coupled with pressure changes induced by sea level variations could subject broad regions to geologically sudden warming events, inducing sediment failure during rapid bursts of clathrate destabilization and methane emission. In scenarios involving multiple, rapid IITPW events or a prolonged IITPW event in which different continental margins experience climatic transition at different times, some or all of the extreme Cambrian carbon cycles could be accounted for by methane release.
3 Elaboration of the IITPW–methane hypothesis
3.1 Perturbations to the carbon cycle
Clathrate destabilization might account for portions of Cambrian carbon cycles either directly, by liberating isotopically light carbon into the global carbon cycle, or indirectly, by inducing second-order effects such as differential weathering and climate change, whose consequences (including changing riverine carbon-fluxes to ocean basins) could perturb the carbon cycle as well.
Furthermore, IITPW events might reasonably be expected to produce these sorts of consequences independently of methane-release, simply by altering paleogeographic positions relative to the Earth's spin axis on a quick and global scale. Thus synergistic factors may lead or lag actual clathrate destabilization and methane ‘bursts’. Future carbon-budget models calculating conceivable methane-release volumes corresponding to the measured Cambrian carbon cycle values must thus be considered as upper-limits only, with all assumptions taken in perspective. Depending upon the mass and extent of the terrestrial biosphere, large methane clathrate deposits could also build up in permafrost layers on land.
At the initiation of a Cambrian inertial interchange event, these portions would move into lower latitudes and experience the start of major sea-level transgression for Laurentia, noted earlier. Both the warming local climate and the incursion of warmer (though still cold) seawater could destabilize permafrost-bound clathrate deposits [67]. Permafrost destabilization might in particular be responsible for the ∼five million year isotopic low observed during the first half of the Nemakit–Daldyn zone at the base of the Cambrian (Fig. 1).
3.2 Clathrate-destabilizing mechanisms
Methane clathrate deposits clearly should respond (stabilizing in some regions, destabilizing in others) to non-eustatic IITPW-induced sea-level change, but other physical ‘triggers’ lie in wait as well. IITPW events should alter ocean-scale thermohaline circulation by changing loci of bottom-water formation and patterns of global heat advection. Any duration of time many millions of years long will experience normal tectonic disruptions in some seafloor settings as well. Even regional-scale eddies – semi-chaotic and unpredictable in nature – can easily drive epistatic changes in, and evolution of, sediment-wave packages. Altogether, methane release by sedimentation regime change must be considered on equal footing with more-straightforward methane release by relative sea-level change in a fair and encompassing hypothesis.
3.3 Temporal independence and long-term susceptibility to methane release
Any IITPW event, regardless of duration, can leave a legacy of eddy instability patterns and sedimentation/erosion changes that are different from one geographic area to the next. Shifting eddies might be ephemeral or they might persist. A particular methane clathrate reserve might lie, untouched, as its sedimentation rate remains constant for some time during or after IITPW only to receive an independent or chain-effect perturbation to sedimentation/erosion further down the road [48].
3.4 Spatial independence and widespread susceptibility to methane release
Finally, during Inertial-Interchange True Polar Wander, continental margins located near the Earth's minimum inertial axis should rotate up to 90°, remaining upon the equator but switching their orientation. Even these shelf-and-slope regions, which move neither pole-to-equator nor equator-to-pole and which experience constant non-eustatic sea level, should experience fluctuating sedimentation and erosion rates due to thermohaline circulation changes.
4 Discussion
4.1 Predictions
Although some previous authors suggest that many of the sharp variations in carbon isotopes observed in the geological record may be related to methane release (e.g., [5,29,43,44,46,66,73,77,83,85,86]), to our knowledge no one has suggested a plausible scenario in which enormous quantities of methane could be stored and released on a timescale and in a repeated way capable of accounting for the Cambrian carbon cycles. The IITPW–carbon hypothesis outlined above may do this. This hypothesis also leads to some testable predictions: (a) barring tectonic overprinting or geodynamic recycling, there should be evidence of sedimentary disruptions in deeper-water facies associated with methane release; (b) there should be significant thermal perturbations associated with the faster carbon oscillations; and (c) if related and not merely coincident with biological diversification during the Cambrian Explosion, there should be a genetic link between these geophysical and geochemical events and natural selection pressures that drive evolution. We consider each in turn (§3.1.1–3.1.3).
4.2 Sedimentary disruptions
Cambrian time is, in fact, littered with examples of sedimentary slumping and intraformational breccias, flat-pebble conglomerates, etc. Some of these sedimentary structures occur on a massive scale, ranging from disrupted bedding horizons in Sardinia [15] and Colorado [81] to the collapse of a ∼60-km length of carbonate platform preserved in present-day British Columbia [102]. To our knowledge, these have not been examined systematically such that they can test the hypothesis suggested here that they might relate to sudden methane escape. Previous interpretations have mainly invoked biological processes (e.g., [99]).
4.3 Temperature fluctuations
By analogy with the LPTM event outlined above, the addition of carbon from methane in quantities large enough to perturb inorganic carbon-isotope ratios (e.g., [28]) ought to produce a measurable temperature increase through simple greenhouse warming – both due to potent atmospheric methane during the volume-dependent but brief period of time immediately following a clathrate destabilization event and during the prolonged, exponential decay of its oxidized CO2 decay-product. Shifts in the carbonate oxygen-isotope record would best test for such an effect. Unfortunately the oxygen signature for most ancient carbonates has been altered by subsequent diagenesis and is only rarely preserved.
4.4 The IITPW–carbon hypothesis as a mechanism for the Cambrian Explosion
In response to the LPTM ‘methane shotgun’ warming pulse, the terrestrial biosphere underwent a massive burst of speciation (e.g., [14]). In effect, the Eocene mammalian radiation is analogous to the Cambrian Explosion except that it consists of one short evolutionary burst rather than a series of repeated bursts.
In the modern world, temperature is the physical parameter that displays the strongest correlation with biological diversity [22]. Biochemical metabolism kinetics are faster at higher temperatures and thus decrease a species' generation time while supporting a robust population more likely to survive sudden or extreme selection pressures. Therefore, in one possible explanation for the Cambrian Explosion, repeated IITPW-induced, methane-release thermal-cycling events incrementally inflate biological diversity, filling and refilling niches with increasingly optimized communities of species. This hypothesis is broadly supported by quantitative models consistent with expansive modern datasets [1] and with paleontological databases [93]. Indeed, as noted previously, many major excursions in Early Cambrian carbon cycles are followed by a clear evolutionary burst in one or more phyletic groups.
In summary, we suggest that the rapid diversification of species and innovation of bodyplans in the Early Cambrian could have been driven by extreme environmental conditions forced by methane clathrate decomposition, induced by an inertial interchange true polar wander event and recorded in the unique sequence of Cambrian carbon cycles.
Acknowledgements
The authors acknowledge Paul Hoffman and an anonymous reviewer; Eiichi Tajika, Ryuji Tata, Keiko Matsuoka, and Tatsuo Oji; Rob Ripperdan, Jess Adkins, David Evans, Adam Maloof, Bob Berner, Mark Pagani, Jim Kasting, Ellis Yochelson, and Osvaldo Ulloa gave helpful and probing comments. This work was supported by the NASA Astrobiology Institute, by NSF-EAR 98-14608 to JLK and by an NSF Graduate Fellowship to TDR.