Version française abrégée
Consécutivement à l'arrêt de l'exhaure minière, l'eau accumulée dans les anciennes mines de fer de Lorraine (France) constituera, à terme, une ressource d'environ 300 millions de mètres cubes. Cependant, lors de l'ennoyage des travaux miniers, la qualité de cette eau se dégrade, ce qui la rend impropre à la consommation [7,9]. Afin de gérer les ressources en eau de cette région, autrefois alimentée en grande partie par l'eau d'exhaure minière [12], il est nécessaire de prévoir à long terme son évolution quantitative et qualitative.
Peu de données spatialisées étant disponibles, c'est une modélisation globale de type « réacteurs en réseaux » (RER) qui a été retenue [5,7]. Un modèle chimique cinétique a été construit de manière à reproduire les réactions entre l'eau et la roche observées lors d'une étude expérimentale en laboratoire [8]. Ce modèle chimique a ensuite été intégré à un simulateur RER pour modéliser le bassin « sud » du Bassin ferrifère lorrain (BFL) [7,9]. Le simulateur permet de reproduire les concentrations observées depuis cinq ans au point de débordement, avec une précision de pour les éléments majeurs (SO4, Na, Ca, Mg et HCO3), donne l'ordre de grandeur de la concentration des éléments secondaires, comme le potassium, et peut être utilisé de manière prédictive. Cependant, les analyses chimiques de l'eau en différents points des réservoirs montrent une hétérogénéité spatiale et des évolutions différentes de ces concentrations, ce dont le modèle ne rend pas compte.
Disposant d'un suivi de la qualité de l'eau dans quatre puits du bassin sud, nous avons construit un modèle semi-distribué afin d'appréhender l'efficacité d'une approche de type RER pour rendre compte de la variabilité spatiale des concentrations.
Le bassin sud a ainsi été divisé en sept secteurs (Fig. 1), cinq d'entre eux contenant l'un des points d'observation (puits et tunnel de débordement). Chaque secteur est modélisé comme une entité de trois mélangeurs représentant chacun les différents types d'exploitation (Fig. 2). Le modèle géochimique utilisé dans chacun de ces mélangeurs repose sur une étude expérimentale et a été décrit dans Collon et al. [7,8]. Le modèle prend en compte les cinétiques de dissolution–précipitation des minéraux, les réactions d'échanges d'ions, la complexation des espèces dans l'eau, l'équilibre avec les gaz O2 et CO2. La valeur des paramètres cinétiques du modèle, définie au cours de l'étude en laboratoire précédente, reste inchangée. Seuls les paramètres caractérisant la nature des roches distinguent les trois types de mélangeurs [7,9]. Dans chaque mélangeur, un encadrement ( et ) de la concentration simulée est calculé. Il s'appuie sur la dispersion observée dans les résultats expérimentaux pour des roches d'un même type [7,9]. La répartition du volume d'eau et des débits d'alimentation dans chaque réacteur est supposée proportionnelle à la surface relative des types d'exploitation qu'ils représentent et qui est calculée à partir des plans miniers digitalisés. Cette distribution se fait avec un répartiteur et un collecteur assure le mélange des flux d'eau sortant des réacteurs. Les entités représentant les sept secteurs sont assemblées en un réseau schématisé sur la Fig. 3. On considère que le débit d'alimentation du bassin minier, , est le résultat de l'infiltration des précipitations. Ce débit a été déterminé par un modèle inverse, basé sur l'analyse des chroniques de données, et permet de reproduire le débit au point de débordement, avec un écart-type moyen de 0,35 m3 s−1 et un coefficient de corrélation [17]. Au temps (1995), les pompages d'exhaure sont partiellement stoppés et l'ennoyage commence. Les secteurs de Droitaumont 1 et 2, les plus profonds, se remplissent en premier en drainant l'eau de l'ensemble du bassin. Puis ils débordent dans Paradis à la concentration , qui déborde à son tour dans Auboué, avec un débit (Fig. 4) et à la concentration . Lorsque le réservoir d'Auboué est plein, il déborde en même temps dans les secteurs de Moyeuvre, Joeuf et Roncourt (Figs. 3 et 4). La répartition du débit sortant d'Auboué entre ces trois zones se fait proportionnellement à la surface de connexion existant. Lorsqu'il est plein, Roncourt déborde dans Joeuf à la concentration , qui déborde à son tour dans Moyeuvre. Ce dernier secteur déborde par le tunnel dans l'Orne à la concentration .

Breakdown of the South Basin into sectors: (D1) Droitaumont 1, (D2) Droitaumont 2, (P) Paradis, (A) Auboué, (R) Roncourt, (J) Joeuf, (M) Moyeuvre; Z1, Z2 and Z3: non-flooded areas.
Division du bassin sud en secteurs : (D1) Droitaumont 1, (D2) Droitaumont 2, (P) Paradis, (A) Auboué, (R) Roncourt, (J) Joeuf, (M) Moyeuvre ; Z1, Z2 et Z3 : zones non ennoyées.
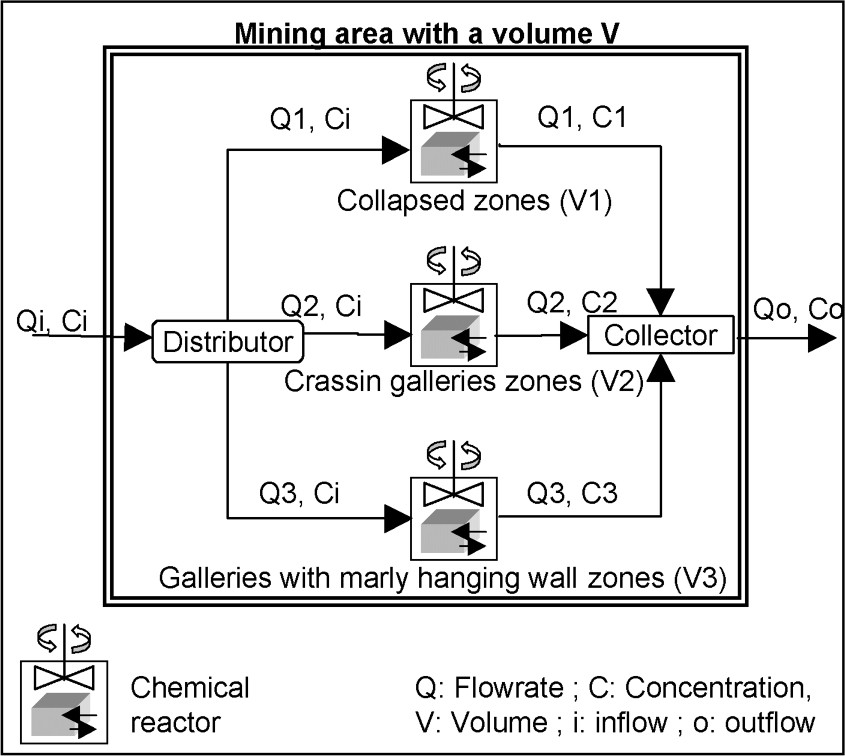
Flow chart of the model adopted for each sector.
Schéma du modèle adopté pour chaque secteur.

Schematic diagram of the hydraulic flow in the South Basin.
Schématisation des flux hydrauliques dans le bassin sud.
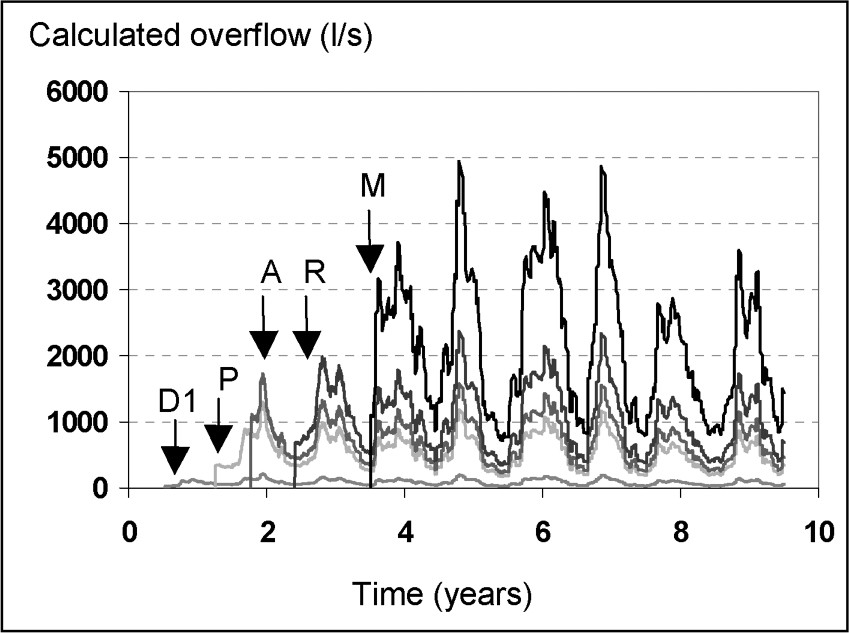
Calculated overflow rate of each sector: (D1) Droitaumont 1, (P) Paradis, (A) Auboué, (R) Roncourt and (M) Moyeuvre.
Débit de débordement calculé pour chaque secteur : (D1) Droitaumont 1, (P) Paradis, (A) Auboué, (R) Roncourt et (M) Moyeuvre.
Notons que chaque secteur est alors caractérisé par (Tableau 1) :
- – sa surface relative : rapport entre sa surface au sol et la surface totale du bassin minier (). Ce paramètre détermine la part des infiltrations qui alimentent le secteur selon l'équation : ;
- – son volume ; l'eau occupant les vides laissés par l'exploitation, on suppose que le volume d'eau total dans le bassin sud (230 millions de mètres cubes) se répartit proportionnellement au rapport entre la surface exploitée dans le secteur () et celle exploitée dans l'ensemble du bassin sud (). On a donc :
Characteristics of the seven areas
Caractéristiques des sept secteurs
Sectors | Symbol | (%) | (m3) |
Droitaumont 1 | D1 | 4.2 | 8.46 × 107 |
Droitaumont 2 | D2 | 3.0 | 6.24 × 107 |
Paradis | P | 16.8 | 4.16 × 107 |
Auboué | A | 8.3 | 2.44 × 107 |
Roncourt | R | 14.6 | 4.8 × 107 |
Joeuf | J | 8.5 | 3.68 × 107 |
Moyeuvre | M | 19.2 | 7.17 × 107 |
Z1 | 14.2 | non-flooded⁎ | |
Z2 | 2.3 | non-flooded⁎ | |
Z3 | 8.9 | non-flooded⁎ |
⁎ Located above the overflow altitude (172 m NGF), these zones will never be flooded.
Quel que soit le point d'observation, le simulateur permet de reproduire le pH et la concentration en HCO3. En effet, ceux-ci sont contrôlés par la pression partielle de CO2 et le rôle tampon des carbonates. De même, l'ordre de grandeur de la concentration en potassium est toujours en accord avec les valeurs mesurées. En ce qui concerne les autres éléments, SO4 (Fig. 5), Ca, Mg et Na, les résultats sont plus variables. Ainsi, les simulations d'Auboué, Roncourt et Moyeuvre sont satisfaisantes. Mais, à l'inverse, les concentrations sont sous-estimées à Droitaumont (particulièrement pour le Na) et à Paradis. Ces différences peuvent s'expliquer de plusieurs façons :
- – à Droitaumont, une simple augmentation de la quantité initiale de sodium échangeable dans la roche permet de corriger nos résultats et d'obtenir une bonne adéquation entre simulation et observation ;
- – à Paradis, l'étude des cartes d'exploitation montre que le puits est situé dans une zone foudroyée où trois couches ont été exploitées. Or, si une continuité d'exploitation apparaît dans la couche grise avec les concessions voisines, l'exploitation des deux autres niveaux est locale. L'eau captée au puits de Paradis ne serait donc pas représentative du mélange de l'eau de tout ce secteur.
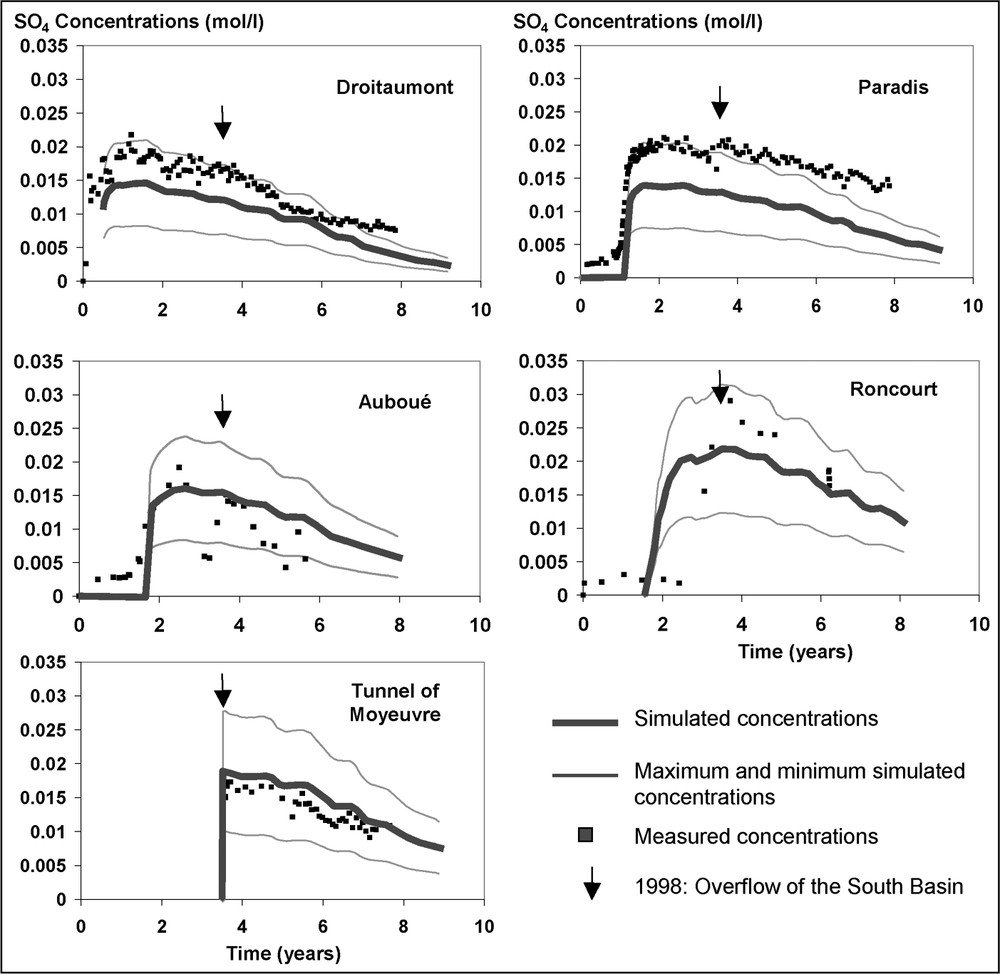
Measured and simulated SO4 concentrations in the four wells and at the overflow point of the South Basin.
Concentrations en SO4 mesurées et simulées dans les 4 puits et au point de débordement du bassin sud.
Le peu de données géologiques spatialisées nous a donc conduits à utiliser une composition minéralogique « moyenne » déterminée en laboratoire, qui ne rend pas complètement compte des variations spatiales à l'échelle du bassin. Par ailleurs, les différentes méthodes d'exploitation et l'existence de concessions minières indépendantes les unes des autres entraînent une distribution des écoulements souterrains non homogène. Pour affiner la modélisation, il est donc indispensable de comprendre et de définir plus précisément les chemins préférentiels d'écoulement dans le bassin.
1 Introduction
In Lorraine (France), the accumulated water in abandoned iron mines will, in the long run, represent a resource of around 300 million square meters. However, when the mine workings are flooded, the quality of this water is lowered, making it inappropriate for human consumption [7,9]. In order to manage the water resources of this region, which was in the past supplied to a large extent by dewatering [12], it is necessary to predict the long-term quantitative and qualitative evolution of this mine water.
Most of the hydrogeochemical modelling studies dealing with mine drainage have been developed for mine tailings, which are similar to porous media (e.g., [2,11,14–16]). In the case of the Lorraine mine reservoirs, for which the data are spatially poorly distributed, we adopted a Network of Chemical Reactors (NCR)-type global approach. Originally developed for process engineering, the association of reactors in series and parallel was used to model the reactive transfer of pollutants in soils [3,13]. This approach was also used to model tailing effluents [1] and the composition of water discharge from flooded mines [6].
An experimental study was carried out on rocks of the Lorraine Iron Basin (LIB) to characterise the nature of the pollution and its probable mineralogical origin. Based on these results, a chemical kinetic model was built that reproduced the water–rock reactions observed in laboratory tests [8].
The LIB is subdivided by faults into three major structural units: the North, the Central, and the South Basins. The modelling was developed for the South Basin. In our previous studies [7,9], the South Basin was represented as a single unit of three parallel reactors. Each reactor corresponds to one of the three types of exploited areas encountered in the mine, in which the water reacts with a type of rock:
- – ‘marly intercalations’ in the collapsed zones;
- – ‘shelly limestone’ locally called ‘crassin’ in the galleries zones;
- – a ‘mixed rock’, composed of 34% of marly hanging wall and 66% of crassin, in galleries within marly hanging wall zones.
The chemical composition of the water at the overflow point of the South Basin is the result of the mixing of water from these three zones.
Each type of reactor is built with the same chemical kinetic model as described in Collon et al. [8]. It includes the dissolution–precipitation kinetics of minerals, the ion-exchange reactions, the aqueous speciation, the equilibrium with CO2 and O2 gases. The values of the kinetic parameters defined formerly in the laboratory experiments are unchanged. The parameters depending on the composition of the rocks distinguish these three types of reactors. In each reactor the maximum and minimum possible concentrations were also calculated, based on the laboratory dispersion of experimental results [7,9].
The model therefore makes it possible to predict the evolution of water quality observed at the overflow of the South Basin for the major elements SO4, Na, Ca, Mg and HCO3 with a precision of ±10% and it gives an order of magnitude for minor elements such as K. The model can be used in a predictive manner; however it gives no information on the spatial distribution of chemical concentrations in the basin groundwater.
Chemical monitoring of water quality is available at four wells of the South Basin. In order to evaluate the accuracy of the NCR in accounting for the spatial variability of the concentrations, we developed a semi-distributed model.
2 Method
2.1 Definition of the areas and their characteristics
The South Basin has been divided into seven sectors: Droitaumont 1 and 2, Paradis, Auboué, Roncourt, Joeuf and Moyeuvre (Fig. 1). Five of them include one of the monitoring points of the basin (wells or overflow). The grey layer [8] wall isohypses are representative of the mine topography [4]. Consequently, the upper limits of Droitaumont 1, Paradis and Auboué follow the grey layer wall isohypse corresponding to the well bottom. Indeed, the NCR approach implies that the calculated concentrations represent those of a point where various waters converge and mix. Consequently, the sectors have been demarcated in order to consider the wells as outlets for each zone. In the southern part, a major structural fault divides the Droitaumont concession into two parts known as parts 1 and 2. These two sectors are not connected, but a cross-cut connects Droitaumont 2 and Paradis. In the northern part, the overflow altitude (172 m NGF) delimits the flooded zone. The existence of structural faults and the exploitation of different layers in this zone mean that it cannot be considered as one group. Consequently, two major faults divide the northern part of the basin into three sectors: Roncourt, Joeuf and Moyeuvre. The Z1, Z2 and Z3 sectors are above the overflow level.
The total volume of water contained in the South Basin (V) and total recharge () were determined with a hydrological model [7,9]. The correlation coefficient between observed and calculated flow rate at the tunnel of Moyeuvre is and the difference between these values are always inferior to 18.5% [17]. The inflow rate is the result of the rainfall infiltration through the Dogger limestone. We assume that each sector receives a part of this recharge, , directly proportional to its relative surface area, (Table 1):
2.2 Conceptual framework of groundwater flow and model construction
At time (1995), the dewatering ceased partially and flooding started. Groundwater flow within the mine reservoir can be divided into six steps (Fig. 3).
(i) At the beginning of flooding, the mine reservoir is empty. Governed by gravity effects, water percolates through the Dogger limestone and recharges the lower sectors: Droitaumont 1 and 2. Droitaumont 2 is only recharged by the rain that falls on its surface area. Conversely, Droitaumont 1 receives the water drained by the upper sectors in addition to its direct rainfall water. Since transfer times through the upper sectors are very short, we consider that the water has a chemical composition similar to that of the Dogger fluids ().
(ii) When these lower sectors are saturated, drained water from the upper sectors no longer recharges them, but now supplies the Paradis sector. Nevertheless, Droitaumont 1 and 2 are still recharged by the rainfall water in proportion to their relative surface area and they both overflow into Paradis with a water composition (Fig. 4).
(iii) When Paradis is saturated, this mine reservoir overflows into the Auboué sector with a flow rate (Fig. 4) and a water composition . Auboué also receives the water drained by Z1, Z2, Z3, Roncourt, Moyeuvre and Joeuf ( composition). Droitaumont 1 and 2 and Paradis are still recharged by the rainfall water in proportion to their relative surface area and the Droitaumont sectors still overflow into Paradis. The units are thus organised into series.
(iv) The Moyeuvre, Joeuf and Roncourt sectors are located at the same depth. Therefore, they start to fill up at the same time, when Auboué is saturated and overflows with a rate (Fig. 4). In proportion to the surface contact that they have with Auboué (estimated on digitalized maps), the overflow of Auboué ( rate, composition) is divided between Moyeuvre (54% of ), Joeuf (45% of ) and Roncourt (0.009% of ), which is connected to Auboué by only one gallery. Each sector is recharged by rainfall water ( composition) and drains the upper unsaturated zones: Z1 for Moyeuvre, Z2 for Joeuf and Z3 for Roncourt. The observed leakage rate is included as an imposed outflow rate for the Moyeuvre sector.
(v) When Roncourt is saturated, it overflows into Joeuf with a flow rate (Fig. 4) and a composition , and then, when Joeuf is saturated, it overflows into Moyeuvre with a flow rate and a composition .
(vi) Finally, when Moyeuvre is saturated, the mine reservoir overflows from the Tunnel of Moyeuvre with a flow rate (; Fig. 4) and a composition .
3 Results and discussion
The simulated concentrations for any observation point are in good agreement with the observed evolution of pH and HCO3. This is due to the buffer action of the CO2 pressure and the presence of carbonates. Moreover, measured K concentrations fall within the range of possible maximum and minimum concentrations calculated by our model. This approach gives estimation for minor elements such as K.
Despite the few observed concentrations available in the Roncourt and Auboué sectors, the results of the model are satisfactory. Indeed, the simulated SO4 (Fig. 5), Ca, Mg and Na concentrations in Roncourt, Auboué and at the overflow point in Moyeuvre are in good agreement with the observed values. Nevertheless, the observed concentrations in Auboué show high fluctuations that correspond to important rainfall. Poor impermeability of the well walls could explain this phenomenon by locally increasing the sensitivity to rainfall. In Roncourt, the delay between the increase in calculated and observed concentrations is due to a presentation artefact: we represent the concentration in this sector while it is being filled, and not when it is saturated, as we do for the others. Since the well is located in the middle level, the sector starts to fill up and shows positive concentrations, even though the well is still above the water level.
In Droitaumont, the Mg simulated concentration is in good agreement with the observed values and the measured SO4 (Fig. 5) and Ca concentrations fall within the range of possible maximum and minimum concentrations calculated by the model. However, the Na concentration is underestimated by the model. This can be explained as a consequence of the uniform mineralogical composition of reactors for all the sectors. Thus, by increasing the initial Cationic Exchange Capacity for Na in the marly intercalations [10], the simulated concentrations agree well with the observed evolution of SO4, Ca, Mg and Na.
In Paradis, the simulated concentrations are underestimated and decrease faster than the observed concentrations for SO4 (Fig. 5), Ca, Mg and Na. This could be explained by a particular position of the well. Indeed, the well of Paradis is located in a collapsed zone where three ore layers have been exploited, but where only one (the grey layer) is connected with surrounding concessions. This can produce a hydraulic confinement of the well, which is not taken into account in the model.
The poor spatially distributed data led us to use an average mineralogical composition determined in the laboratory. This approximation does not completely account for the spatial variations at the scale of the basin. Moreover, the different exploitation methods and the existence of independent mining concessions involve a non-homogeneous distribution of groundwater flow. Therefore, in order to reproduce the concentrations in the different monitored wells, the spatial organisation of the flows have to be specified.
4 Conclusion
The accumulated water in the abandoned iron mines of Lorraine (France) will represent, in the long run, a resource of around 300 million m3. However, the flooding of the mine workings involves a degradation of the groundwater quality, making it inappropriate for human consumption. Taking care of the lack of both hydrological and geological data, a semi-distributed modelling approach is required in order to predict the long-term quantitative and qualitative evolution of this resource.
Global modelling based on an NCR approach (Network of Chemical Reactors) was, therefore, used to simulate the evolution of the chemical composition of water in four mining wells of the South Basin. In this model, the South Basin is represented with a network of seven sectors. Each of them is made up of three reactors corresponding to the three types of exploitation encountered in the mine.
Although encouraging, the results show the need to specify the spatial organisation of water flow in the reservoir in order to reproduce the evolution of chemical concentrations in the different monitored wells.
Acknowledgements
This work is part of the research programme carried out by GISOS (‘Groupement de recherche sur l'impact et la sécurité des ouvrages souterrains’ – Research Group for the impact and safety of artificial underground cavities).