1 Introduction
Contrary to non-volcanic passive margins (or else sedimentary passive margins, SPM) volcanic passive margins (VPM) are narrower and associated with the accretion, during continental break-up, of a thick magma crust [70,82,83] (Fig. 1). This magma crust is composed of heavily intruded continental crust covered with flood-basalts and tuffs, seismically imaged as strongly reflective Seaward Dipping Reflectors Sequences (SDR; [4,36,62]). Underneath the intruded transitional crust, high-velocity () seismic zones (HVZ) are usually interpreted as bodies of underplated mafic to ultra-mafic magma [38,43,82].
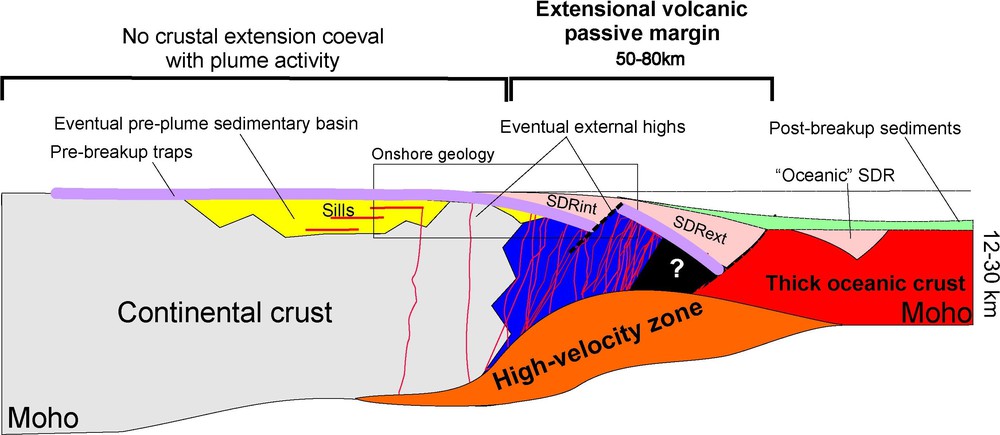
Across-strike section of a volcanic passive margin. The presence of internal sedimentary basins is not the rule. SDRint and SDRext: respectively, internal and external seaward-dipping lavas and volcanic projections (i.e. ‘Seaward-Dipping Reflectors’ in offshore studies).
Coupe schématique transverse d'une marge passive volcanique. L'existence de bassins sédimentaires en position interne n'est pas obligatoire. SDRint et SDRext : prismes de laves inclinées vers l'océan, en position interne ou externe (Seaward-Dipping Reflectors).
Whereas the structure of SPM is known down to the Moho due to their transparency to seismic waves in seismic-reflection experiments [7,53], the internal structure of MPV is poorly constrained by MCS studies due, notably, to strong impedance contrasts within the SDR. In addition, accurate comparison between seismic and deep boreholes data in seaward-dipping wedges of basalts, outline that seismic reflectors may be parallel to the true lava flows, but do not image any particular lava flow by themselves [25,67,68]. Also, seaward-dipping lava formations may exist without being expressed by any seismic reflection [25,67,68]. Actually, our present knowledge of VPM incomes from wide-angle seismic surveys coupled with inversion of potential data (e.g., [5,74]) and from detailed onshore surveys in exposed VPM [10,31,42,52]. Nowadays there is evidence that a majority of passive margins belong to the VPM-type [25] (Fig. 2) justifying the strong international effort in their study.
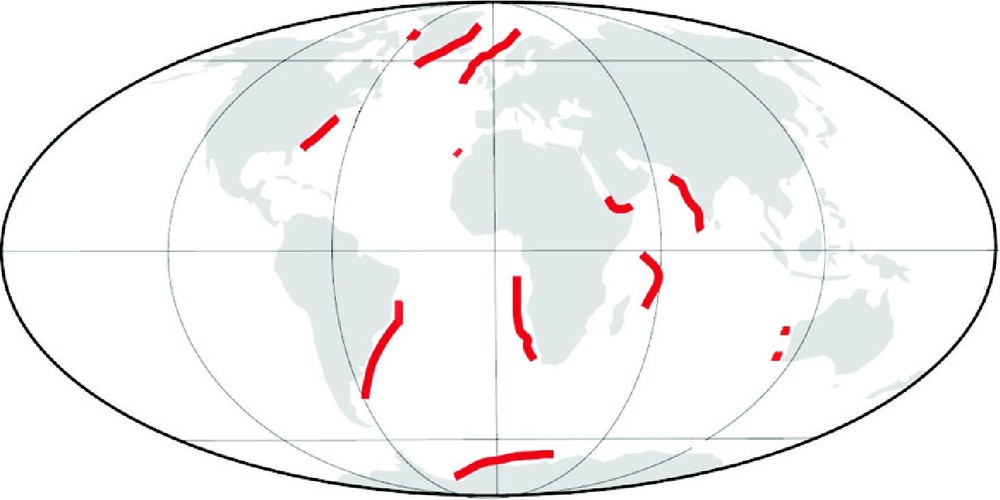
Worldwide distribution of volcanic passive margins (in [14], after [16]).
Distribution mondiale des marges volcaniques (dans [14], d'après [16]).
2 Origin of VPM
2.1 Extension and VPM
VPM and SPM have distinct origins. They are respectively inherited from volcanic and sedimentary rifts. Sengor and Burke [76] first introduced the concepts of active and passive rifting from geological observations to account for the differences between, respectively, volcanic and non-volcanic rifts. These former concepts were largely used and later updated. Fig. 3 summarizes some of the common concepts (or/and possibly, misconceptions) concerning both active and passive modes of extension.
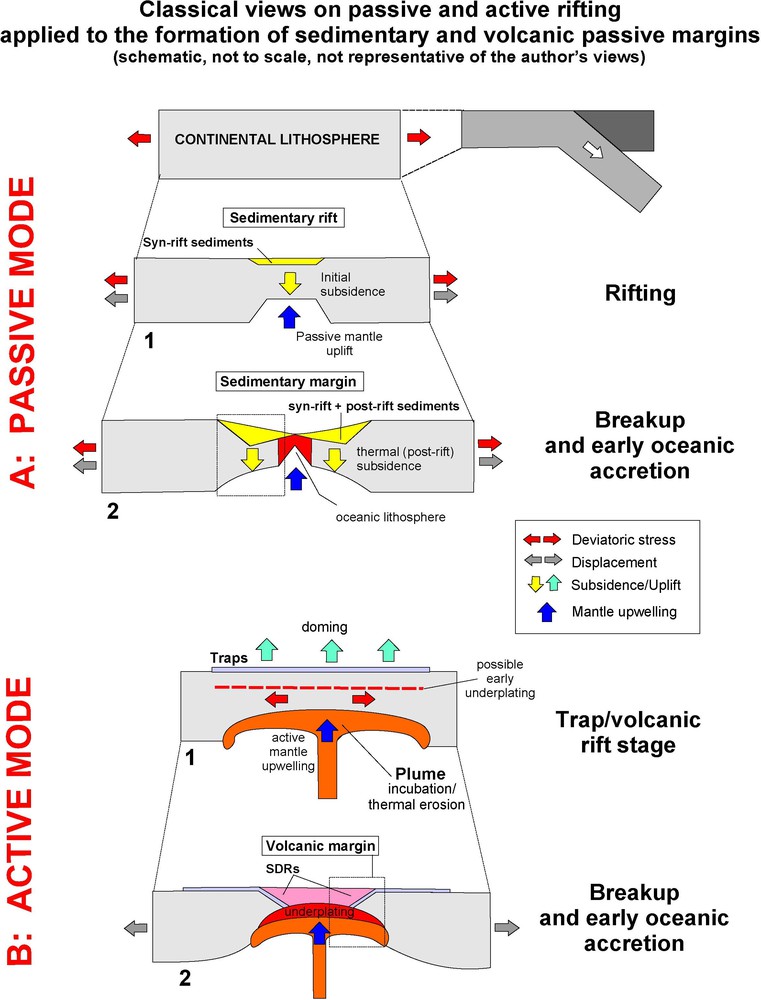
Passive (A) and active (B) mechanisms of lithosphere extension. From a synthesis and simplification of numerous studies that followed [76].
Mécanismes passifs (A) et actifs (B) d'extension lithosphérique (d'après une synthèse de très nombreux travaux, dont [76]).
Plate tectonics drives lithosphere extension in the passive model (Fig. 3A). Passive upwelling of buoyant sub-lithospheric mantle occurs beneath the thinned area [59,76]. Subsidence – and sedimentation – occurs both during the syn-rift (initial subsidence) and post-rift (thermal and long-term subsidence) stages [59]. When occurring, mantle melting is a late consequence of the lithosphere dynamical stretching [60,83]. Mantle melting is strongly enhanced if the sub-lithospheric mantle is hot [83].
According to the purely active model (Fig. 3B), a hotter-than-normal mantle (for example, mantle plume) thermally thins the bottom of the lithosphere [27,35,64]. Adiabatic mantle melting occurs with rates increasing with time, due to continuous decrease in mantle pressure as the lithosphere thins. The two stages may be distinguished (Fig. 3B): (1) flood-basalt stage, coeval with very small crustal extension and, (2), break-up stage associated with VPM edification [17]. Break-up is consecutive to tensional forces associated with lithosphere thinning [79]. Uplifting or apparent isostatic equilibrium predates and accompanies VPM development [15,19,71,81]. Thermal subsidence occurs after break-up, with amplitude depending upon the thickness of the newly-formed igneous crust and upon the persistence of the thermal anomaly.
Although useful and founder, the Sengor and Burke's concepts are weakened by new mechanical and geological evidences.
Mechanically, the lithosphere cannot be regarded anymore as a rigid conductive entity limited at its base by a horizontal isotherm [1,22,41,61]. Especially, no more confusion should exist between mechanical and thermal lithosphere. Considering realistic P- and T-dependent viscosity, the base of the thermal lithosphere is unstable and small-scale convecting, in the presence [27,34] or not [61] of a thermal anomaly. In addition, lateral variations in lithosphere thickness (i.e. age or thermal state) can influence the pattern of mantle flow, especially at craton edges [46]. Thus, a non-consideration of small-scale mantle dynamics in the processes of continental break-up and mantle melting seems irrelevant.
Geologically, many VPM developed in areas that were previously submitted to far-field extension so contradicting Fig. 3B's purely active model [83]. Although discontinuous in time and space, lithosphere extension initiated in the Northeast Atlantic during the Carboniferous and continued until the Palaeogene (Fig. 4) [85]. Sudden break-up and VPM development occurred only during the Eocene (C25–C24) following the covering with traps of a large uplifted area during the Palaeocene (Fig. 4B). The Palaeocene basalts (ca 2 km in thickness) or coeval sediments sealed the Late Cretaceous extensional faults [29,32]. This Northeast Atlantic evolution is mainly based on the extensive study of the Vıring margin, in Norway (Fig. 4A and C) (e.g., [32]). Across this margin, the syn-sedimentary extension apparently migrated ‘oceanward’ with time until the final Eocene break-up associated with VPM edification (Fig. 4C) [32,80]. Stretching rates during the Mesozoic were low (for example, during the −170 to −95 Ma period, [80]), whereas the syn-magmatic break-up seems a very sudden phenomenon (see [36,70] and §3). This Northeast Atlantic case suggests that a thermal weakening of the lithosphere was necessary to induce the complete and focalised continental break-up, whereas previous low-rate syn-sedimentary extension would solely provoke lithosphere hardening and lateral basin migration. Concerning the latter point, one may remark that the inward strain migration proposed for the Vıring margin [80] (Fig. 4C) is opposite, in sense, to the outward migration predicted from theoretical rifting models with low-rate stretching and lateral heat diffusion [48].
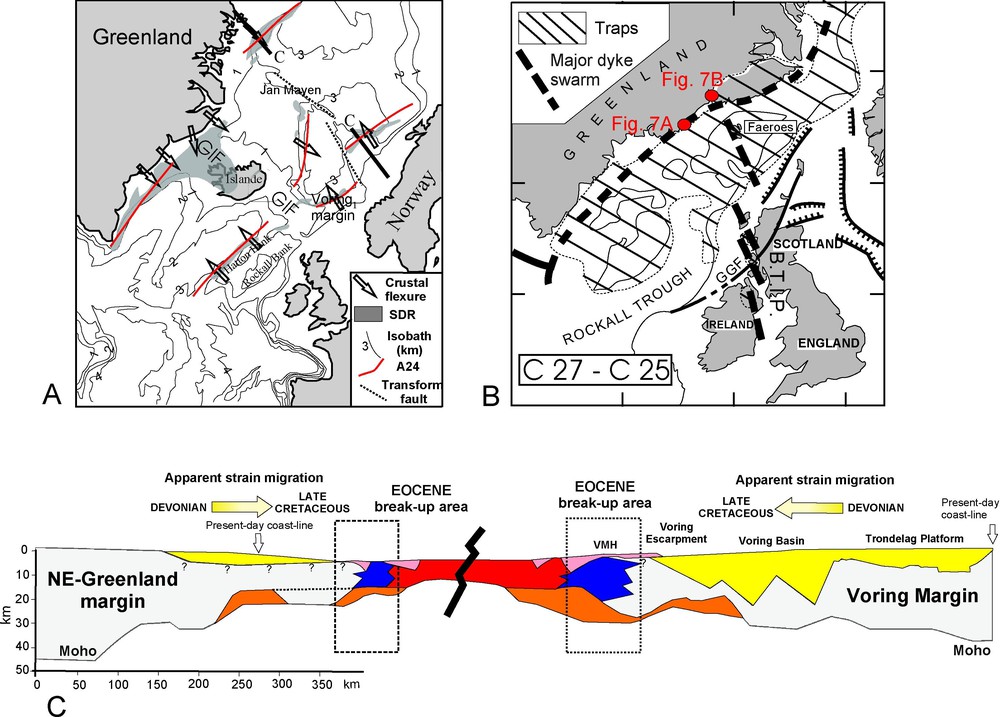
Conjugated volcanic passive margins in the Northeastern Atlantic. (A) Present-day location of VPM (GIF: Greenland-Iceland-Faeroe aseismic ridge). (B) North Atlantic Province during the Palaeocene (trap stage, C27–C26). (C) Crustal across-strike sections of the conjugated Vıring/NE-Greenland margins). Colours have the same meaning as in Fig. 1. After [29,74] and J.I. Faleide, pers. commun.
Marges passives volcaniques conjuguées de Vıring et du Nord-Est du Groenland. (A) Position des marges et localisation du profil C (GIF : ride asismique Groenland–Islande–Faeroe). (B) Province Nord-Atlantique au Paléocène (mise en place des traps, C27–C26). (C) Coupes des marges conjuguées Nord-Est du Groenland/Vıring. Même code couleur que pour la Fig. 1. D'après [29,74] et J.I. Faleide, pers. commun.
The setting of a number of VPM (e.g., [5,39,83]) at the edge of previous sedimentary basins (Fig. 1) questions the (strange) causality between plate extension and the upwelling of deep mantle plumes commonly invoked by a number of authors to explain VPM magmatism (e.g., [35,83]). It also suggests that far-field extensional stresses are in any case necessary to form a rift, whatever it is sedimentary or volcanic. However, if far-field stresses are necessary, there are strictly no evidences that a previous lithosphere stretching is a sine qua non condition for VPM development. VPM may indeed develop in areas that were not submitted to significant lithosphere stretching and thinning before the trap emplacement (see, for example, the Afar case [17]). Based generally on the sole Vıring example, some authors have integrated the very long-term evolution and architecture of sedimentary basins that may surround VPM (Fig. 1) as part of the development and architecture of VPM themselves. This has led to frequent misunderstanding on the tectonics of these specific margins [30].
2.2 Magmatism
VPM belong to the so-called Large Igneous Provinces (LIP). Magmatism at VPM predates (plateau basalts emplacement), accompanies (SDR, HVZ, intrusions within the transition crust) and, also, post-date continental break-up. Enhanced mantle melting following break-up is an important characteristic of most VPM. It is expressed, during oceanic accretion, by a thicker-than-normal oceanic crust including, in some cases, ‘oceanic’ SDR and HVZ (Fig. 1). For example, the oceanic crust may reach up 40 km in thickness in Iceland and along the Greenland–Iceland–Faeroe aseismic ridge (GIF; Fig. 4A) and oceanic SDR are described in both cases [49]. As the oceanic crust thickness is directly linked to mantle potential temperature, thick oceanic crust postdating break-up is the most convincing argument for a hotter-than-normal mantle during VPM formation [60,83].
A particular case is the U.S. ‘East Coast’ margin. This margin is clearly of VPM type (with SDR and HVZ) [43]. However, it is adjacent to a normal-in-thickness oceanic crust and characterized by an important post-rift subsidence, two features usually related to SPM. Hypothetically, the thermal anomaly preceding and accompanying the continental break-up [84] vanished as soon as the beginning of oceanic accretion.
Volume estimations of magma products at VPM [16,24,38,83] are made difficult due, notably, to incertitude in the significance of HVZ and in the volume estimation of eroded plateau basalts. During VPM edification, the rate of magma production is thought to be bracketed between 1 and 10 km3 yr−1. It would exceed 25% of the total oceanic ridge annual production [16,51]. During the Phanerozoic, volcanic passive margins are thought to be associated with an average accretion rate of 0.4 km3 yr−1 of new igneous transitional crust (Fig. 1) [83]. This can be compared to the upper-bound estimate of ca 1 km3 yr−1 for the global rate of continental crust accretion at the same period [69].
The aim of this short review paper is not to present a comprehensive review of the igneous petrology and geochemistry at VPM (see, for example, [57]). In the North Atlantic, the (generally) enriched basalts that form traps (Figs. 3B and 4B) are (often) first of alkaline and then tholeiitic type [73,78]. Most of the traps ( in volume?) emplaced during the Palaeocene (ca −62 to −60 Ma [37]). The local occurrence of high volumes of early picritic lavas [50] is used as a supplementary argument for high mantle temperatures [52]. The SDR (Figs. 3B and 6) emplaced between −56 and −53 Ma. There are generally tholeiitic, the internal wedges being contaminated by continental crust [70].
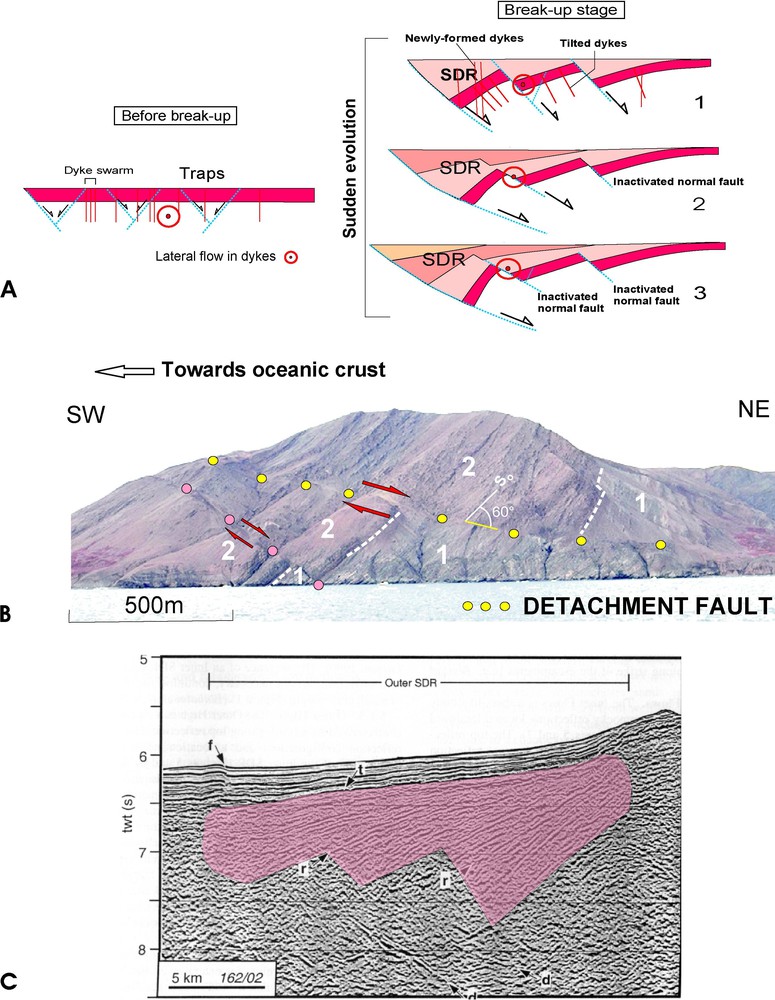
(A) Formation of SDR at VPM [30]: SDR are syn-magmatic roll-over flexures accommodated by continentward-dipping normal faults. (B) Example of tilted continentward-dipping normal fault (yellow dots) in the internal SDR of SE-Baffin Bay (Svartenhuk Peninsula, see [31]). Note also the reactivation of a dyke as a secondary normal fault during the seaward-tilt of the lavas and projections (red dots). (C) Outer SDR-prism west of Australia [68]. Note the structure analogue to (A) and the existence of continentward-dipping faults (d) here interpreted as magma-injected normal faults.
(A) Mode de formation des SDR, interprétés comme des anticlinaux en roll-over développés au-dessus de failles à pendage vers le continent [30]. (B) Exemple de SDR interne, avec faille basculée à pendage vers le continent (points jaunes). Noter la réactivation d'un dyke comme faille secondaire (points rouges) pendant le basculement de la série, constituée de laves et surtout de projections volcaniques. Svartenhuk, marge volcanique du Sud-Est de la baie de Baffin [31]. (C) Prisme SDR externe de la marge Ouest-Australienne. Noter la structuration analogue à celle de (A) et la présence de failles profondes à pendage vers le continent (d), interprétées comme étant injectées de magma [68].
The controversial issue of the mantle reservoir(s) that feed LIP is the topic of a large number of papers (e.g., [1,3,18,57,73]). To understand better the interaction between the hot mantle and the in-extension lithosphere, it is crucial to gather information on the depths of mantle melting during the process of VPM formation. Independent trace-elements geochemistry [44,73,78] and P-waves velocity modelling of the HVZ [38] suggest that the adiabatically melting hot mantle rises actively upward during VPM formation through the basal lithosphere. Holbrook et al. [38] propose that the rate of active mantle penetration through the lithosphere could be up to ten times as large as the half-rate of lithosphere stretching. This postulated behaviour of a low-viscosity and buoyant active mantle should be regarded in connection with the theories on small-scale convection at the base of the lithosphere [30,40]. This sets the fundamental (although neglected) issue of the detailed topology of the mantle melting areas beneath LIP.
In LIP the area covered with traps is usually considered as coincident at depth with the melting head of a mushroom-like mantle plume. This idea is based on a too simple representation of the way basic magmas migrate from mantle to the Earth surface. The model in Fig. 5 for MPV magma growth is based on the following geological and geophysical grounds [30]: (1) both traps and SDR are fed by a limited number of dyke swarms which are parallel to the rift-zones (Fig. 4B) (e.g., [12,13,29]); (2) most micro-seismological and geological data (e.g., [14]), as well as mechanical models [56], point to the fact that dykes in rift-zones are fed laterally from upper-crustal magma reservoirs in which the magma differentiates; (3) flood-basalts may flow up to several hundred kilometres from their feeder dyke [75]. The model in Fig. 5, close to the one proposed at slow-spreading ridges [55], suggests that crustal magma reservoirs feeding traps and SDR are localized over melting mantle zones corresponding to the tops of small-scale convecting cells. The undifferentiated mafic magma extracted from the mantle at small-scale active cells would (1) migrate upward to the crustal reservoirs, (2) differentiate within the crust (note the probable strong continental contamination at this stage) and (3) inject laterally upward in dykes in the transitional crust (still contaminating). This margin magma segmentation model implies a correlation between the wavelength of small-scale convection and the spacing of magma centres. This point has recently been demonstrated [12]. Another consequence of the model is to postulate periodic variations in the total melt thickness (HVZ and SDR) in the transitional crust, a point that is hereafter discussed.
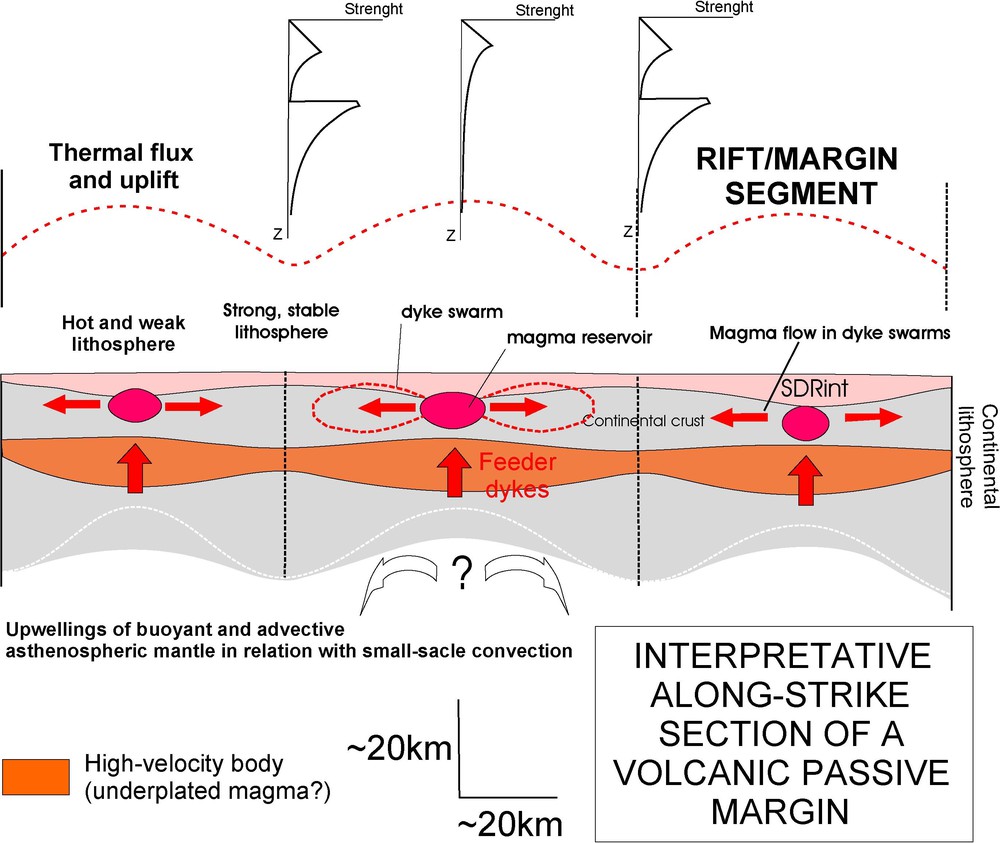
Along-strike growth model for volcanic passive margins. From [30], modified.
Modèle de croissance magmatique des marges passives volcaniques en section longitudinale. D'après [30], modifié.
3 Structure and development of VPM
3.1 Crustal extension at VPM
A common issue concerning VPM is the localisation and expression of crustal extension [25,70]. Many authors used the Palmason's model for Icelandic flexures [65] to claim that SDR at VPM were isostatic in origin and fed symmetrically from a single and permanent central accretion axis [62].
More recent onshore [10,30,31,42] and offshore investigations [25,32,33,68,77] have pointed out that SDR are syn-magmatic roll-over tectonic flexures whose development is controlled by major continentward-dipping normal faults (Fig. 6). VPM often display two or more SDR wedges (Fig. 1), the inner one being clearly ‘continental’ (Fig. 1). One of this inner SDR wedge outcrops in western Greenland (Svartenhuk Peninsula) [30] (Fig. 6B). Eastern Greenland, the eroded transitional crust located beneath the inner-SDR outcrops continuously, forming the famous ‘coastal crustal flexure’ (Fig. 7A). These examples among others [10] allowed reconstructing with some details the tectonic evolution of the inner part of VPM in 2D across-strike sections. During traps emplacement (Fig. 6A), quite small-extension (<1%) is undertaken by rift-parallel normal faults, often conjugated, and dyke swarms. The following formation of SDR (Fig. 6A) is a sudden phenomenon (see hereafter) associated with a strong lithosphere necking. The SDR development is accommodated by dyking (Fig. 7A), but also by arrays of continentward-dipping normal faults, rotated and progressively inactivated as the SDR wedge develops oceanward (Fig. 6A and B, [30]). Note that the development of the ‘oceanic’ SDR in Iceland is quite similar [20]. The flexed continental crust located beneath the inner SDR is considerably dilated by margin-parallel dykes that feed overlying traps and SDR (Figs. 1 and 7). Early dykes that fed traps, initially sub-vertical, are tilted oceanward of the finite angle α of the seaward crustal flexure, whereas dykes that are emplaced during the flexure (feeding the SDR) crosscut the former and display various dips from 90° (latest dykes, no tilt) to () [9,10,31,42] (Fig. 7A). It is interesting to note that the maximum the flexure is (tectonic extension), the maximum of horizontal magma dilatation is observed (up to 80% locally).
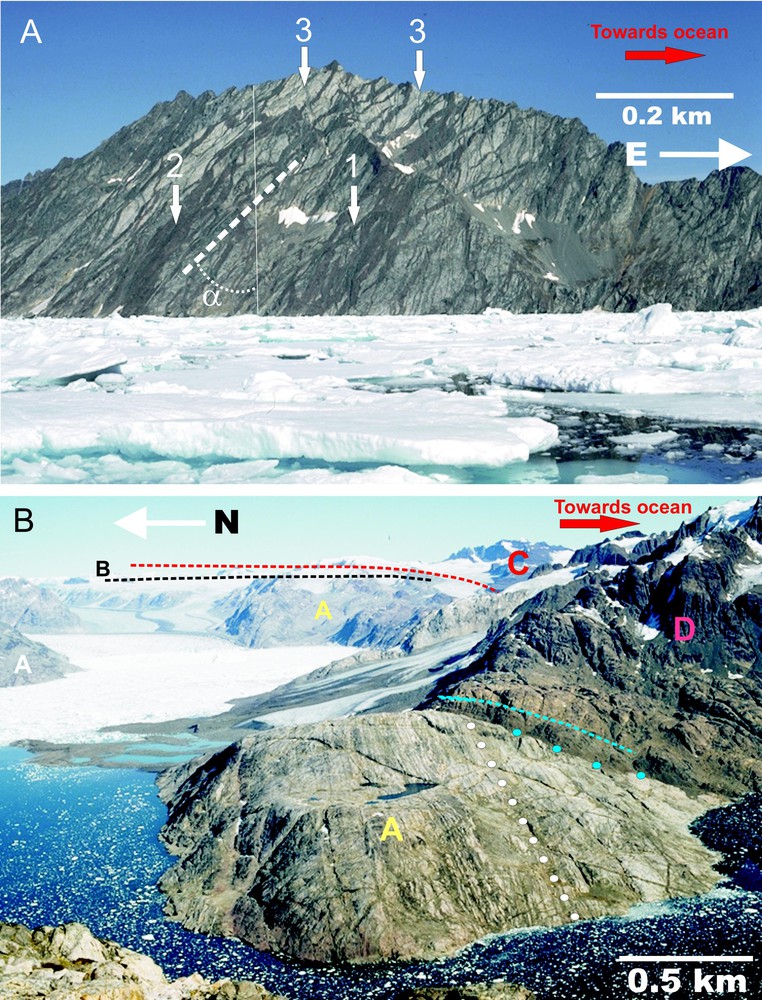
(A) Uplifted and eroded transitional crust (East-Coast flexure, Greenland, location in Fig. 4B). Numerous basaltic dykes, mostly of Eocene age [52], cut the Precambrian continental crust. In the picture's area, the crust is flexed seaward of an angle α of ca 55°. Most dykes were initially injected as vertical intrusions. Generation-‘1’ dykes were injected before the development of seaward crustal flexure. They are now dipping continentward of an angle 90°−α. Dykes ‘2’ were injected during the crustal flexure (they display various dips between 90°−α and 90°). The latest generation (3) forms vertical intrusions that crosscut generations 1 and 2 and postdate the crustal flexure. (B) Example of central igneous intrusion (Skaergaard, D) that belongs to a larger group of syenite/gabbroic intrusions in eastern Greenland around the Kangerlussuaq area (location in Fig. 4B). The intrusion presents a clear magma foliation (blue dashed line), parallel to its brittle contact (blue dots) with the transitional crust (A). The Precambrian transitional crust is cut by numerous dykes that are sub-vertical (not tilted), because this area is some kilometres inward the seaward-coastal flexure (see Fig. 1). In the background, one can observe seaward flexed Palaeogene lavas (red dashed line) overlying Cretaceous sedimentary formations (approximate base: black dashed line).
(A) Exemple de croûte de transition (croûte continentale précambrienne injectée de dykes basaltiques), située sous les SDR érodés (niveau d'érosion : −2 à −4 km sous l'ancienne surface topographique). Côte sud-est du Groenland, altitude de la falaise : 85 m (localisation, Fig. 3A). Une majorité de dykes, principalement d'âge Éocène [52], se sont mis en place sous forme de filons verticaux. La croûte présente une flexure finie α vers l'océan (au niveau de ce site : α=55°) attestée par le basculement passif des dykes de génération « 1 » de l'angle fini α de la flexure. Les dykes de génération « 2 » se sont mis en place pendant la flexure (ils recoupent les dykes « 1 » et présentent des pendages variés, compris entre (90°−α) et 90°. Les dykes de génération « 3 » recoupent systématiquement les dykes 1 et 2, sont sub-verticaux et post-datent la flexure. (B) Exemple d'intrusion hypovolcanique dans la croûte de transition (A, injectée de dykes). Nord-Est du Groenland, Skaergaard (localisation sur la Fig. 4B). L'intrusion gabbroïque (D), litée (tiretés bleus), n'est qu'un élément de petit volume d'un ensemble d'intrusions de très grande taille-essentiellement composées de syénites et de gabbros (système intrusif de Kangerlussuaq). À l'arrière-plan, on distingue les traps et SDR Paléocène–Éocène (C), ainsi que des formations sédimentaires d'âge Crétacé (B). La position de la base des laves et du sédimentaire (respectivement, tiretés rouges et noirs) est approximative. Noter l'attitude subverticale des dykes tertiaires dans le Précambrien (points blancs) qui s'explique par le fait que l'on se trouve en position interne relativement à la flexure crustale (SDRi), développée vers la droite de la photographie. Noter également le contact froid de l'intrusion centrale, parallèle au litage magmatique (respectivement, pointillés et tiretés bleus) et l'existence de quelques dykes gabbroïques dans l'encaissant, qui sont parallèles au contact. Se référer à la Fig. 1 pour comprendre l'organisation générale des formations A à D.
3.2 3D crustal structure
The dyke swarms that feed traps and SDR display en-échelon pattern in horizontal plane view. Each swarm is clearly centred on magma reservoirs (at least, on the central hypovolcanic complexes located over them) [9,14]. A similar organisation is also met along the ancient and recent Icelandic rift zones [20]. In addition, finite extensional strain (SDR-related fault-controlled crustal flexure) also increases towards these reservoirs [30,31]. Although they remain 2D across-strike views, some MCS studies have also suggested the relationships between SDR wedges and large intrusive complexes [38,47].
Mainly from geological evidences, Geoffroy [30], followed by Callot [12], has defined the concept of individual rift segment for VPM (Figs. 5 and 8). Each VPM segment (∼50–70 km in length) is centred on a crustal magma reservoir. These reservoirs have a crustal-scale importance (e.g., [5]) and are met in all LIP [12]. Their surface expression is a large polygenic volcano whose activity may span 1 Ma and more. Due to tensional stress concentration, dykes initiate at the edges of the reservoir and propagate laterally away from it, in the trend of the regional maximum horizontal stress (that is, parallel to the rift axis) [21]. When dykes cross-cut the ground surface they give rise to flood basalts and monogenetic cones alignments (Fig. 8). The maximum finite magma dilatation is met around the magma reservoirs. In addition to their control on magma segmentation, normal faults seem to initiate and propagate around these reservoirs (Fig. 8). As for dyke injection, the stress pattern around the reservoirs may indeed mechanically favour the initiation and consecutive propagation of normal faults [21]. Concerning this latter point, it should however be remarked that the main mechanical control for crustal faulting is more probably dominated by the weak strength of the lithosphere beneath the reservoirs (see §4). Although some of them may abort, the magmatotectonic segments usually exist from the flood-basalt stage [21] to the break-up stage. Their length seems dependent upon the thermal state of the lithosphere [8,23]. With the increase of lithosphere stretching, the individual magmatotectonic segments mechanically interact to form a continuous line of break-up [13]. Because the magma centres are not always aligned, the rift axis may present a sinuous (or ‘zigzag’) pattern as observed in western and eastern Greenland [12,31].
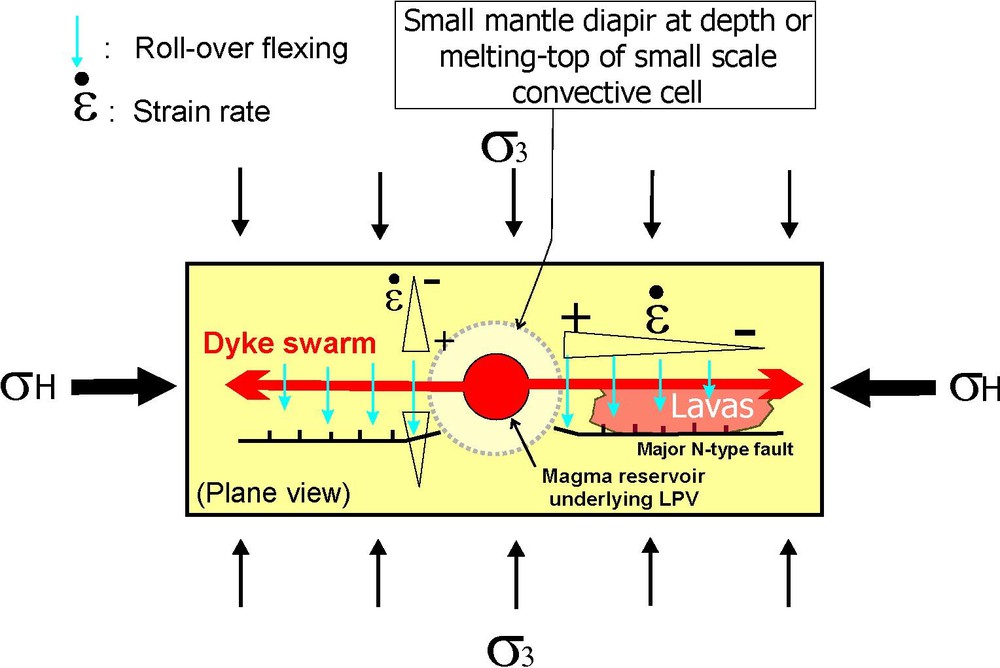
Volcano-tectonic rift segment [21,30]: relationships between major normal faults, fault-controlled elastic flexure and magma reservoir. Note the increase in both finite strain (tectonic flexure – blue arrows – and dilatation through dyking) and strain rates towards the magma reservoir. Most dykes are injected laterally away from the reservoir, in the trend of the maximum horizontal stress , and feed at distance the lava field if they intersect the topographic surface [14].
Segment de rift volcano-tectonique [21,30] : relations entre failles normales, flexure élastique et réservoir magmatique. Noter l'augmentation (1) de la déformation finie (flexure crustale et dilatation par injection de dykes) et (2) des taux de déformation, vers le réservoir magmatique. Le magma s'injecte latéralement dans les dykes à partir du réservoir magmatique, dans la direction de , et alimente le champ de laves lorsque les dykes recoupent la surface topographique [14].
Offshore and closer to the ocean-continent transition, VPM often present along-strike a gravity and magnetic segmentation. The shortest wavelengths are comparable to those measured at adjacent oceanic ridges [6,74]. Although this physical segmentation is certainly related to the magmatotectonic one observed onshore, these anomalies have not yet been fully inverted in a satisfactory 3D crustal model. Gravity segmentation could be linked to the presence of the mafic/ultramafic crustal magma reservoirs themselves (at least in the most internal part of the margins), and/or else to along-strike variations in thickness of the magma crust (SDR, HVZ). The strong along-strike magnetic anomaly along the VPM transition crust (e.g., ECMA, [6]) is possibly due to the remnant magnetization of the SDR and intrusions within the transitional crust and, hypothetically, to the part of the HVZ located above the Curie temperature [66,74]. That this coastal magnetic anomaly varies along-strike seems an indication of along-strike periodic variations in total melt thickness at VPM. In the Northeastern Atlantic, industrial seismic data confirm along-strike thickness variations of SDR [Gernigon, pers. commun.] in a way quite similar to Fig. 5 along-strike model. In addition, the HVZs top surface would display a complex 3D corrugation beneath the Northeastern-Atlantic sedimentary basins [32,74].
3.3 Strain rates at VPM
Two methods may be used to infer finite strain and strain rates at VPM. Syn-magmatic continental extension is bracketed in time between traps emplacement and the first magnetic anomaly. Finite extension can be estimated from the thickness ratio between non-extended and post-extension transition crust (Fig. 1). This method supposes evidently that the bulk extension at VPM is pure-shear [59], a non-resolved issue. It also poses the tricky problem of the crust-mantle boundary location near HVZ. A more accurate method was proposed by Lenoir et al. [52]. They estimated lower-bound values of horizontal extension rates along the East Greenland coastal flexure by dating pairs of cross-cutting pre-flexure and post-flexure dykes (Fig. 7A). The results (strain rates probably over 10−14 s−1) demonstrate (at least locally) the correctness of a former proposition by Hinz from SDR study [36]: VPM traduce a catastrophic break-up of the lithosphere.
4 Farther on the significance of VPM
The most striking points that characterize VPM (by comparison with SPM) are (1) the coincidence between melting and strain-concentration areas (Figs. 5 and 8) and (2) the particular geometry of finite crustal extension (syn-magmatic seaward-dipping crustal flexures associated with continentward-dipping faults; Figs. 1 and 6).
4.1 The soft-point model
Lithospheric rheological models that combine ductile (power-law) and brittle rheologies (friction) predict at the least two high-strength mechanical layers within the continental lithosphere: the upper crust and the upper lithosphere mantle [11,48]. As discussed here before, at volcanic rifts and margins (1) melting seems to be strongly localized within the buoyant mantle and (2) extension seems to initiate and concentrate over these melting areas. The postulated hot and shallow small-scale mantle rises that feed crustal reservoirs certainly weaken thermally and locally the lithosphere columns located other them (Fig. 5). Callot et al. [13] tested this hypothesis by the analogical modelling of a 4-layer lithosphere submitted to passive extension and containing local viscous inclusions in place of the rigid mantle lithosphere (silicon in place of sand in the upper lithosphere mantle) (Fig. 9) [13]. These viscous anomalies aimed at reproducing the rheological effects of localized stronger thermal gradients (Figs. 5 and 9). These experiments clearly demonstrate that extension within the crust initiates above the deep viscous anomalies, thus defining individual rift segments. With increasing extension, the rift segments become wider and longer and connect. A continuous and linear rift-zone finally appears when soft points are aligned whereas a ‘zigzag’ rift pattern (as observed along some VPM, see §3) appears when soft areas are not aligned [13].
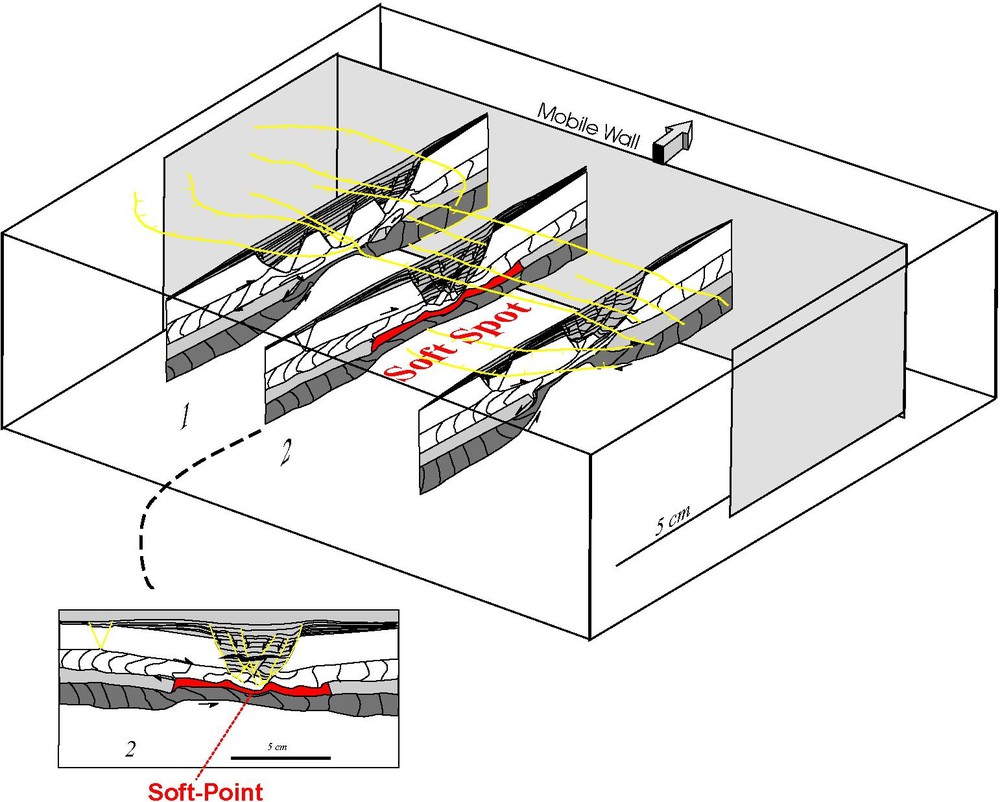
Four layers analogical modelling of the ‘soft-point theory’ for continental break-up (see text and [13]).
Modélisation analogique 4 couches de la théorie des « points mous rhéologiques » de Callot et al. (cf. texte et [13]).
4.2 Tectonic interpretation of crustal flexures
In the brittle upper crust, the sense of dip of normal faults seems in direct dependence upon the sense of shear at the interface between the ductile crust and the brittle crust [11,26]. At SPM, immediately beneath the fragile crust, this ductile shear is thought to be oceanward, as is the dip of the major normal faults that accommodate extension in the overlying upper crust [7,53] (Fig. 10A). In analogical models of lithosphere necking [11], a deeper conjugated continentward shear accommodates the overall bulk pure shear of the lithosphere (Fig. 10A).
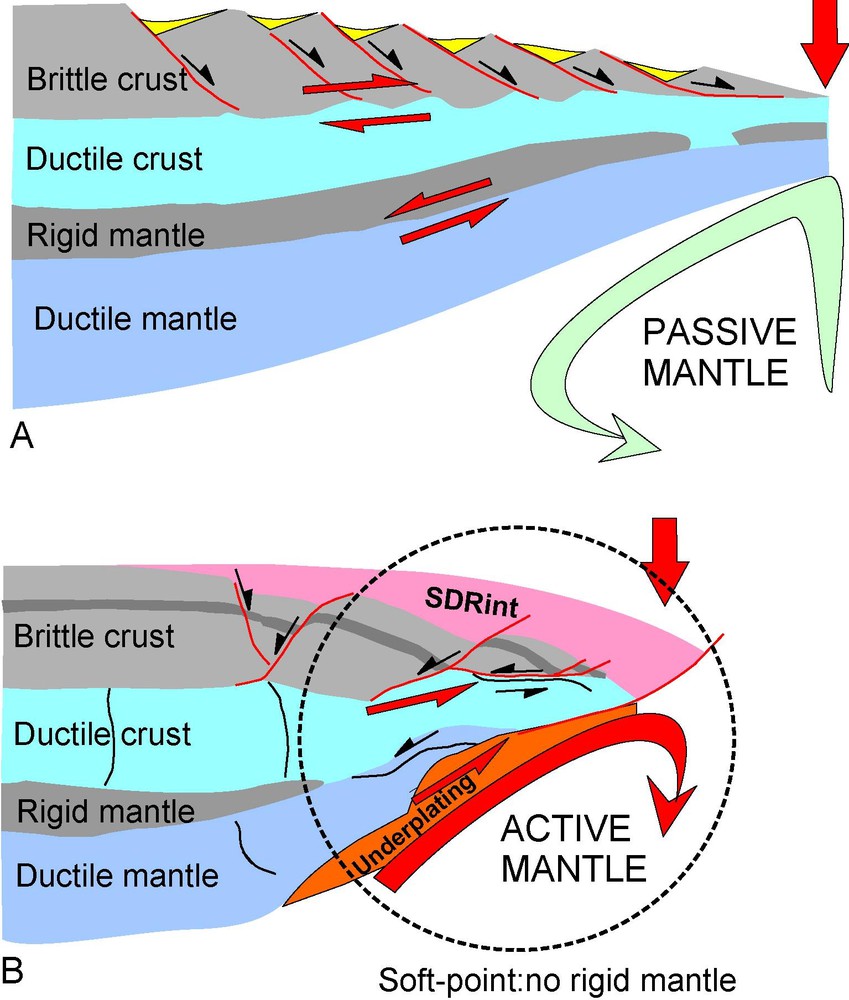
Proposed relationships between mantle dynamics (A: passive, SPM; B: active, VPM) and lithosphere extension. These figures are inspired from published [11–13,28] and unpublished analogical and numerical experiments. A: Sedimentary passive margin, B: volcanic passive margin. Further explanations in the text. Vertical red arrow: break-up area and first oceanic crust.
Relation possible entre la dynamique du manteau (A : passive, B : active) et la déformation en extension de la lithosphère continentale au niveau, (A), d'une marge sédimentaire et (B) d'une marge volcanique. Ces figures sont inspirées de résultats de modélisations analogiques et numériques publiées [11–13,28] ou non. Explications dans le texte, codes couleurs identiques aux autres figures pour la croûte (cf. Fig. 1). Flèche rouge verticale : zone de rupture lithosphérique et d'océanisation.
At VPM, due to higher thermal-gradients, strain seems to be concentrated over a 2-layer rheological lithosphere (see above, Figs. 5 and 9), i.e. a brittle crust overlying a ductile lithosphere. Contrary to SPM, but following the same reasoning, the continentward sense of dip of the major discontinuities that control the development of SDR could be associated mechanically with a large continentward shear at the brittle-ductile crust interface (Fig. 10B). We suggest that the active and melting asthenosphere (see §2 and 3), which is flowing upward and laterally oceanward, could drag along the overlying ductile lithosphere (ductile crust and mantle lithosphere) towards the break-up area, thus explaining the sense of shear at the bottom of the rigid upper crust (Fig. 10B; [28]). The magma that invades the break-up zone (including the HVZ body) would form by decompression melting of the upward-laterally flowing asthenospheric/lithospheric (mixed?) mantle. This postulated transient and high-speed flow of the buoyant upper mantle is perhaps a consequence of complex combination of transient ‘break-up suction’ [45,63] and active small-scale convection [61] along the break-up axis (Fig. 10B) (see also [2]).
5 Conclusion
One can hardly state that VPM and SPM represent end-members of a single process of lithosphere break-up. VPM present distinct structural and evolutionary features. Although some volcanism may exist at SPM during the syn-rift and/or post-rift stages [58,72], although some SPM also are narrow margins [54], VPM present a combination of quite general specific features such like (1) significant igneous crust accretion, (2) narrow (‘necked’) margins, (3) seaward crustal flexures and continentward-dipping faults, (4) no subsidence during stretching. Both their along-strike magmatic and tectonic segmentation and the evidences for the active behaviour of a hot and buoyant mantle, suggest that VPM share more characteristics with slow-spreading oceanic axes [6,55] than with SPM. Probably do VPM represent in the Earth's geodynamics one of the best case-example of the importance of sub-vertical flows of buoyant mantle in governing, in addition to plate-tectonic forces, the processes of plate break-up.
Acknowledgements
This work is supported by the GDR Marges (contribution No. 2058), by the European Science Foundation (program Euromargin–Eurocore EMA01), and by the IPEV (program No. 290). Thanks to colleagues and students who contributed to these projects. Thanks to the anonymous reviewers and to Laurent Gernigon and Hilary O'Donnel for their quick look on the English version of this manuscript.
1 Une version détaillée de cet article en français peut être téléchargée en format pdf sur le site http://www.univ-lemans.fr/~lgeof/public.htm/CRAS.