1 Introduction
Soils would be in a sustainable state if constituents were removed at the same rate as they are produced. Unfortunately, physical erosion rates of agricultural soils worldwide exceed the natural soil production rate, as well as the natural erosion rates under native vegetation (Montgomery, 2007; Quinton et al., 2010; Wilkinson, 2005). Physical erosion also affects the dynamics of the biogeochemical cycling by removing organic matter, and nutrients such as N, P, and K from the cropped areas (Quinton et al., 2010). However, the human impact on both physical and chemical weathering is not fully assessed, and models taking into account both aspects still need to be developed (Brantley, 2008).
Terrestrial plants play a major role in the evolution of landscapes and in global geochemical cycles (Amundson et al., 2007). Roots and associated microorganisms produce organic acids and chelates, which enhance the chemical weathering of soil minerals and the release of elements in soil solutions (Berner et al., 2005; Lucas, 2001). Enhanced chemical weathering provides pools of elements, which are taken up, stored and recycled at various rates by plants, and are ultimately released in streams at a rate up to 18 times the rate calculated for bare watershed (see review in Berner et al., 2005).
In agriculture, optimised yields are often combined with limited element recycling because of harvesting and subsequent total or partial export from the field. Although at a global scale N and P inputs by fertilizers are larger than exports by plants (Quinton et al., 2010), implying that these two elements do not experience soil depletion, little is known about the other elements taken up by plants that are not brought back to the soil through fertilization or plant recycling. This is particularly the case for Si (Clymans et al., 2011; Struyf and Conley, 2012), the most common element on Earth, which is found in large concentrations in many grass crops (Guntzer et al., 2011). The extent of agriculture impact on the biogeochemical cycle of Si is crucial for maintaining high crop yield (Ma and Takahashi, 2002), as well as for improving the prediction of the global change through modelling (Laruelle et al., 2009). Here we address the question of the perturbation of the phytoliths pool in soil, which has been recognized as a key parameter in the Si biogeochemical cycle in natural ecosystems since the pioneer work of Bartoli (1981, 1983).
2 Si weathering in natural ecosystems
The weathering of silicates, which cover 70% of the continents, is closely linked to the global cycle of carbon (Berner and Kothavala, 2001). In the oceans, dissolved Si mainly originates from the weathering of silicate minerals at the surface of the continents (Struyf et al., 2009). During the Precambrian, oceanic Si was controlled by tectonic, weathering and hydrothermal inputs (Robert and Chaussidon, 2006). Since the Phanerozoic, the oceanic silica levels have been dominated by organic reactions through silica-secreting organisms (Tréguer et al., 1995). Falkowski et al. (2004) showed that the diversification of grasses and diatoms coincided during the Paleogene most likely because Si mobilized by terrestrial plants provided an enhanced nutrient delivery to the oceans that was favorable to radiation of diatoms. On the continents, the role played by plants in the global cycle of Si is less well understood but it is striking that the annual fixation of Si in plants rivals that fixed in the oceanic biogeochemical cycle (Conley, 2002; Loucaides et al., 2010). In addition, Struyf and Conley (2012) recently calculated that the annual fixation of dissolved Si into terrestrial vegetation is 10 to 40 times more than the yearly export of dissolved and biogenic exports to the coastal zone.
Although silicon is not essential to plants, it is considered beneficial because it alleviates both biotic and abiotic stresses (Epstein, 1999; Guntzer et al., 2012). Plants take up Si from the soil solution as silicic acid, which, after translocation to the shoots, is polymerised through water evaporation and precipitated as phytoliths (Alexandre et al., 1997). Phytoliths are composed of opal A (XRD-amorphous phase) particles although some authors have suggested the presence of opal CT (Deelman, 1986). Contrary to many nutrients, Si cannot subsequently be redistributed within the plant, because the phytoliths remain insoluble until plant remains return to the soil and start decomposing. Consequently, Si is mostly located in shoots and concentrations vary between < 0.1 and 10% Si in shoot dry matter (Epstein, 1999).
In natural ecosystems, dissolved silicon (DSi) occurs mainly as Si(OH)4 originating from the dissolution of primary minerals. DSi may follow several paths according to the biogeochemical environmental conditions: adsorption on the surface of oxides, precipitation as amorphous silica or clay minerals, release to the streams by gravity or uptake by plants. Particulate Si can reach the soil through dust fallout or litterfall as phytoliths (see review in Cornelis et al., 2011a; Sommer et al., 2006; Street-Perrott and Barker, 2008). However, the dynamic of soil Si sinks is still not well understood (Struyf and Conley, 2012), and the mechanisms that control the Si cycle are probably time-dependent, with time lapses ranging from seconds (adsorption) to millennia (clay formation), with intermediate periods corresponding to the residence time of phytoliths.
Several studies have evaluated the fluxes of Si between plants, soil solutions and soil minerals mostly in natural forested ecosystems (Table 1a). They show that fluxes vary according to the age and the type of forests, and that plants absorb a significant fraction of dissolved Si, which originates from litterfall decomposition i.e. from phytoliths dissolution. This fraction is usually mostly recycled, while export to streams is limited. The amount of silicon released by weathering of soil minerals other than phytoliths is highly variable and depends on vegetation type.
Flux de Si estimés ou calculés dans quelques écosystèmes naturels (a) et agrosystèmes (b).
Plant cycling | Output | References | |
kg Si ha−1 yr−1 | |||
(a) Natural ecosystems | |||
Tropical forest (young) | 7 | 7–21 | Meunier et al., 2010 |
Tropical forest (mature) | ca. 50 | 11–26 | Lucas et al., 1993 |
Tropical forest (mature) | 58–76 | 16 | Alexandre et al., 1997 |
Temperate deciduous forest | 22 | ɛ | Bartoli, 1983 |
Temperate coniferous forest | 5 | 26 | Bartoli, 1983 |
Temperate coniferous forest | 36 | 5.0 | Gérard et al., 2008 |
Temperate coniferous forest | 14.27 | 17.26 | Markewitz and Richter, 1998 |
Douglas fir | 30.6 | 1.1 | Cornelis et al., 2010 |
Norway spruce | 43.5 | 0.7 | Cornelis et al., 2010 |
Black pine | 2.3 | 9.4 | Cornelis et al., 2010 |
European beech | 23.3 | 6.0 | Cornelis et al., 2010 |
Oak | 18.5 | 6.7 | Cornelis et al., 2010 |
Short natural grass (Arik) | 22 | 0.5 | Blecker et al., 2006 |
Mixed natural glass (Smokey Valley) | 56 | 0.3 | Blecker et al., 2006 |
Grasslands (Santa Cruz) | 12–48 | 5–19 | White et al., 2012 |
Tall natural grass (Konza) | 67 | 6.3 | Blecker et al., 2006 |
Savanna (Ivory Coast) | 127 | 9 | Alexandre et al., 2011 |
(b) Agrosystems | |||
Non grass crop | 3–8 | ASi+ DSi? | Vandevenne et al., 2011 |
Fodder grass | 32–53 | ASi+ DSi? | Vandevenne et al., 2011 |
Cereal crop (rice) | 270 | ASi+ DSi? | Desplanques et al., 2006 |
Cereal crop (rice) | 500 | ASi+ DSi? | Makabe et al., 2009 |
Cereal crop (wheat) | 37–113 | ASi+ DSi? | Vandevenne et al., 2011 |
Cereal crop (wheat) | 94 | ASi+ DSi? | Guntzer et al., 2012 (estimates) |
Cereal crop (wheat) | 20 | ASi+ DSi? | Guntzer et al., 2012 (Rothamsted data) |
The quantification of the phytoliths pools is still not calibrated and two methods are currently used for the determination of the amorphous Si pools (ASi): (1) a non-destructive technique based on heavy liquid extraction (Alexandre et al., 1997; Kelly, 1990); (2) a destructive technique using alkaline solutions which allows for the quantification of the amorphous Si from silicates (DeMaster, 1981; Saccone et al., 2007). Both techniques are able to extract as well other biogenic silica particles, such as diatom frustules, and pedogenic silica from secondary minerals but only the physical extraction can verify whether the proportion of non-phytolith particles is negligible or not.
Few studies have investigated the Si cycle in natural grasslands (Table 1a): for a range of grasslands, Blecker et al. (2006) calculated that a large pool of Si was recycled through litterfall while output and weathering were limited. The impact of grasslands on Si recycling appears to be of the same order of magnitude as forests because the lower biomass is offset by larger Si concentration and turnover. Indeed, grasses are known to accumulate more Si in their shoots than do most species with concentrations above 1% Si in DM (Hodson et al., 2005; Ma and Takahashi, 2002). However, Blecker et al. (2006) also calculated that grasslands had a twofold increase on mineral dissolution over forests suggesting that Si biocycling may influence mineral weathering to a greater extent in grasslands as compared to forests.
The experimental studies performed by Fraysse et al. (2006, 2009, 2010) show that phytoliths are one of the most important soluble sources of Si as compared to other soil minerals despite their low concentration in soils. Indeed, among all soil minerals, phytoliths have the highest dissolution rate, except at pH < 3–4. The dissolution of 0.1 to 1% of the phytoliths pool may be enough to explain the values of dissolved Si found in surface waters (Fraysse et al., 2010). The high solubility of phytoliths can even control the DSi concentration in rivers as suggested for Hawaiian streams (Derry et al., 2005). However, tracing the proportion of biogenic versus lithogenic origin of riverine DSi in still not well constrained (Struyf and Conley, 2012).
3 Phytoliths in cultivated land
There is little data available on Si mass balance in agrosystems (Table 1b). Most information is related to the amount of Si taken up by plants, but data on both dissolved Si export and chemical weathering are missing. Except for non-grass crops (Table 1b), Si uptake by crops exceeds the uptake by forests and natural grass ecosystems. The five most cultivated crops worldwide, namely sugar cane, maize, rice, wheat, and barley belong to the grass (Poaceae) family (Guntzer et al., 2012). The other main difference between cultivated and natural ecosystems is that through harvesting the plant remains do not necessarily return to the soil, which increases the export part of the Si mass balance (Struyf et al., 2010; Vandevenne et al., 2011). Matichenkov and Bocharnikova (2001) have estimated that the amounts of Si taken up by crops and carried out by rivers are of the same magnitude (ca. 200 Mt Si yr−1). Assuming that phytoliths are the only source of Si for crops, a simple calculation considering an exportation of 50–100 kg Si ha−1 yr−1 (Table 1b) and an initial pool of Si from phytoliths of 1 t Si ha−1 (Bartoli, 1983; Gérard et al., 2008), shows that under temperate climate and initial forest setting, the phytoliths pool would be depleted within a few decades. When comparing data from various ecosystems (Table 2), it appears that the phytolith pools vary according to soil types (Clarke, 2003) and vegetation cover. ASi in agrosystems is of 800–13 458 kg ha−1, which is within the range of values obtained for natural forests (7–31 262 kg ha−1) but largely lower than the range of values obtained for natural grasses (18 000–71 100 kg ha−1). ASi is larger in natural grass ecosystems than in natural forest ecosystems, because grasses are the world largest plant accumulators of Si (Hodson et al., 2005).
Stocks de Si phytolithique dans le sol, calculés pour différents écosystèmes naturels et agricoles.
Vegetation cover | Soil type | Soil phytoliths kg Si ha−1 |
Soil depth cm |
Methods | References |
Natural vegetation | |||||
Tropical forest (young) | Leptosol | 7 | 1 | Vegetation | Meunier et al., 2010 |
Tropical forest (mature) | Ferrasol | 17000 | 50 | Calculation | Lucas, 2001 |
Tropical forest (mature) | Spodosol | ?? | 50 | Calculation | Lucas, 2001 |
Temperate deciduous forest (Housseras) | Dystric Cambisol | 450 | 50 | Physical extractiona | Bartoli, 1983 |
Temperate coniferous forest (Biffontaine) | Spodosol | 1400 | 50 | Physical extraction | Bartoli, 1983 |
Temperate coniferous forest | Dystric Cambisol | 852 | 120 | Calculation | Gérard et al., 2008 |
Tropical forest (mature) | Ferrasol | 17100 | 50 | Physical extraction | Alexandre et al., 1997 |
Mixed continental forest | Spodosols | 17410 | 50 | Na2CO3b | Saccone et al., 2008 |
Boreal-nemoral mixed forest | Typical Cambisol | 31262 | 85 | Na2CO3 | Clymans et al., 2011 |
Mixed natural grass (Smokey Valley) | Mollisol | 67000 | 50 | Physical extraction | Blecker et al., 2006 |
Short grass (Arik) | Arid Mollisol | 31000 | 50 | Physical extraction | Blecker et al., 2006 |
Tall grass (Konza) | Wet Mollisol | 18000 | 50 | Physical extraction | Blecker et al., 2006 |
Natural grass | Mollisol | 55000 | 20 | Physical extraction | Borrelli et al., 2010 |
Acacia stand following natural grass | Mollisol | 71100 | 20 | Physical extraction | Borrelli et al., 2010 |
Grasslands (Santa Cruz) | Loamy Mollisols | 4841–8780 | 15–25 | Physical extraction | White et al., 2012 |
Savanna | Ferrugineous soil | 3300 | 10 | Physical extraction | Alexandre et al., 2011 |
(Sub)arctic wetland | Peatland | 5607 | 50 | Na2CO3 | Struyf et al., 2010b |
Freshwater marsh vegetation | Mudflats | 15000 | 30 | Na2CO3 | Struyf et al., 2006 |
Agricultural land | |||||
Cereal crop (rice) | Fluvisol/Reductisol | 800 | 25 | Physical extraction | Desplanques et al., 2006 |
Cereal crop (wheat) | Luvisol | 6508 | 50 | Na2CO3 | Guntzer et al., 2012 |
Cereal crop (wheat) | Luvisol | 7259 | 50 | Physical extraction | Guntzer et al., 2012 |
Pasture | Typical Cambisol | 12757 | 85 | Na2CO3 | Clymans et al., 2011 |
Arable land (mixed cereals) | Typical Cambisol | 13458 | 85 | Na2CO3 | Clymans et al., 2011 |
a Physical extraction of phytoliths using densimetric separation (Kelly, 1990).
b Alkaline extraction using Na2CO3 (DeMaster, 1981).
Studying Si soil profiles offers an insight into the dynamics of phytoliths. In natural forests or grass systems (Alexandre et al., 1997, 2011; Blecker et al., 2006; Borrelli et al., 2010; Saccone et al., 2007), ASi is larger at the surface due to input through litterfall. ASi decreases rapidly below the surface but remains constant at depth, which probably reflects different pools of phytoliths, including a more soluble pool at the surface and a more resistant pool at depth (Alexandre et al., 1997). At depth, however, ASi may increase again due to phytoliths translocation (Cornelis et al., 2011b; Fishkis et al., 2010).
Two recent studies, conducted under similar climatic conditions, have reported differences in ASi storage according to land use. The first study (Clymans et al., 2011) used the Na2CO3 extraction method and found that the distribution of ASi followed the same trend in forested and agriculture lands with maximal ASi concentrations in the topsoils. This study also showed larger ASi pools under forest (Table 2). The origin of ASi depletion in agriculture lands was attributed to a reduced ASi replenishment due to a systematic removal of crop residues. However, no information was given on the history of agricultural practices to support this interpretation. In addition, the Na2CO3 extraction technique used to quantify ASi has not been calibrated so far for phytoliths and may either not be adapted to dissolve all types of soil phytoliths (Cabanes et al., 2011) or may give excessive values when inorganic amorphous solids are present (Sauer et al., 2006).
The second study (Guntzer et al., 2012) used a combination of alkaline and physical extractions to test the ASi depletion hypothesis in a 153 y straw export experiment: the Broadbalk continuous winter wheat (Triticum aestivum L.) experiment at Rothamsted Research, UK. This study showed that Si concentration increased with depth, an opposite trend to that found by Clymans et al. (2011). The general trend was a decrease in ASi concentration during the first 30 years of experiment, followed by a low and steady concentration till present. Both alkaline and physical extractions provided similar results, pointing out to a putative ASi depletion in cultivated soils due to straw export (Fig. 1).

Decrease of ASi (in % Si in DW) over time in the topsoil of the long-term continuous wheat experiment with straw export at Broadbalk (Rothamsted Research, UK); white circles are ASi obtained by Na2CO3 extraction and black triangles are ASi obtained by physical extraction using by ZnBr2; redrawn from Guntzer et al. (2012).
Diminution des concentrations en ASi (en % Si du poids sec de sol), au cours du temps, dans l’horizon de surface de l’expérience de monoculture de blé avec exportation des pailles à Broadbalk (Rothamsted Research, Royaume-Uni). Les cercles représentent les données obtenues par extraction au Na2CO3 et les triangles les données obtenues par extraction physique au ZnBr2. Repris des données originales de Guntzer et al. (2012).
Comparing ASi profiles from these wheat field soils with profiles from similar soils left to return to forest, it appears that 120 years of forest did not significantly modify Si concentration profiles except for the topsoil of the Geescroft Wilderness, which had not been limed and was characterized by a drop of pH down to 4.4 and the presence of unweathered phytoliths. This is in accordance with the limited amount of amorphous Si found in forests under temperate climate and with thermodynamic data indicating a slower phytoliths dissolution rate at low pH (Fraysse et al., 2006), as experienced at the Geescroft Wilderness.
In polder paddy fields of increasing age (Kojima, Japan), Ma and Takahashi (2002) observed a decrease of soluble Si over time in the soil profile, which was correlated to a decrease in grain yield and Si concentrations in rice (Oryza sativa L.) shoots. A pH decrease was also observed in the whole soil profile that might be partly responsible for the decrease in soluble Si. Ma and Takahashi (2002) concluded that both pH decrease and plant uptake were responsible for the decrease in soluble Si concentration in soil. Unfortunately, soluble Si refers to immediately phytoavailable Si and does not include phytoliths and thus cannot be compared to the Broadbalk results. Following results of the Clymans et al. (2011) and Guntzer et al. (2012) experiments, we speculate that changes in soil pH might modulate the ASi pool through increase or decrease of the dissolution rate of phytoliths. While a decrease in pH may reduce the dissolution rate of phytoliths, it may increase that of other soil crystalline silicates (Fraysse et al., 2009). The contribution of soil silicates other than phytoliths to phytoavailable Si may therefore increase with decreasing pH. Indeed, the amount of Si in soil silicates was found to be 30 times larger than the Si phytoliths pool in natural grassland (Blecker et al., 2006), or even 50 times larger than the Si phytoliths pool in the Broadbalk experiment (Guntzer et al., 2012).
4 Preliminary results from a decade export experiment.
A decrease in the soil ASi content, as observed in the Broadbalk experiment, may be related to changes in soil characteristics such as pH through continuous cultivation and not only to straw export. In order to test if the incorporation of straw to soils increases the soil ASi pool, we sampled three plots from a Luvisol at the Arvalis-Institut du végétal station in Boigneville (France), which were cultivated for 36 years with winter wheat (Triticum aestivum L.) and maize (Zea mays L.) from 1970 to 1997, and with sugar beet (Beta vulgaris L.), wheat and pea (Pisum sativum L.) from 1998 to 2006 (Fig. 2a). During 12 years, one of the plots had half of the wheat straw exported after harvest while maize straw was totally exported (CR+E). In the second one, straw was always returned to the soil (CP+R). Both plots were ploughed down to 25 cm and sown classically, while a third one was directly seeded and straw was always returned to the soil (noted as DS+R). In the later, only the first 5 cm of soil were homogenized. Soil samples were taken from the first 20 cm in the conventional ploughing settings (CP+R and CP+E) and the first 5 cm in the direct seeding setting (DS+R).
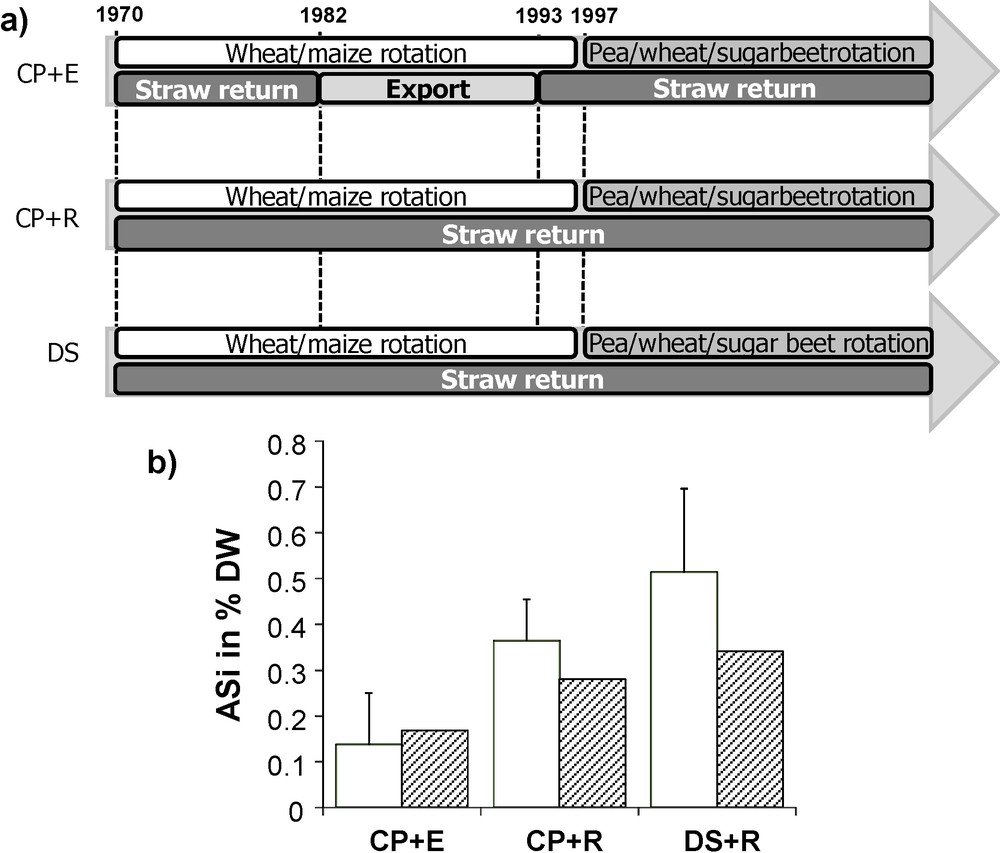
Straw restitution experiment at Boigneville, Arvalis experimental station, France. a) Scheme of the crop rotation; b) effect on ASi concentrations using Na2CO3 extraction (white) and ZnBr2 extraction (black) in the topsoil. CP = classical ploughing down to 25 cm; DS = direct seeding; E = straw export and R = straw return.
Expérience de restitution de paille à Boigneville, station expérimentale d’Arvalis, France. a) Déroulé des actions ; b) effet sur les concentrations en ASi (en blanc, extraction alcaline par Na2CO3 et en noir = extraction physique par ZnBr2) dans les horizons de surface. CP = labour classique jusqu’à 25 cm ; SD = semis direct et R = restitution.
All soil samples were air-dried and passed through a 2-mm sieve before Si extraction. We used two techniques for ASi analyses: heavy liquid extraction using zinc bromide (ZnBr2) alkaline extraction by 0.1 M Na2CO3, at 85 °C (Guntzer et al., 2012). The fractions extracted with the non-destructive method were weighed and mounted on slices for optical microscope inspection (Leica DM RXP) in order to characterise their state of conservation and to detect the presence of non-phytolith amorphous silica particles. We calculated the content of Si in phytoliths after checking that the analysed extract was only composed of phytoliths, and assuming that the water content of phytoliths is 10% (Bartoli, 1981), which gave a proportion of 42% of Si in the fraction extracted by zinc bromide. Three or four replicates of each sample were performed with the Na2CO3 procedure while the heavy liquid extraction was conducted only once per sample.
Results indicated an increase in both ASi measurements (Fig. 2b) in the CP+R as compared to the CR+E setting. The effect was still measurable 15 years after the experiment had been terminated. ASi measurements gave also larger values under direct seeding due to concentration of phytoliths in the first five centimeters: although the total phytoliths amount within the whole profile was probably as much as that of the CP+R, this concentration at the surface clearly evidence the importance of straw return or litterfall at the soil surface.
We also observed that the straw restitution influenced the phytoliths types observed in the soils. Indeed, the soil samples that had straw restitution have more (34% and 42%) elongate phytoliths originating from epidermal long cells (observed in wheat stems) and less short cell phytoliths (rondels and trapeziform polylobates) than the soil sample that had straw exportation (20%) (Fig. 3). Also, we did not observe dendriform phytoliths typical of wheat inflorescences (Ball et al., 1993), or cross-shaped and saddle-shaped phytoliths typical of maize (Piperno, 2006).
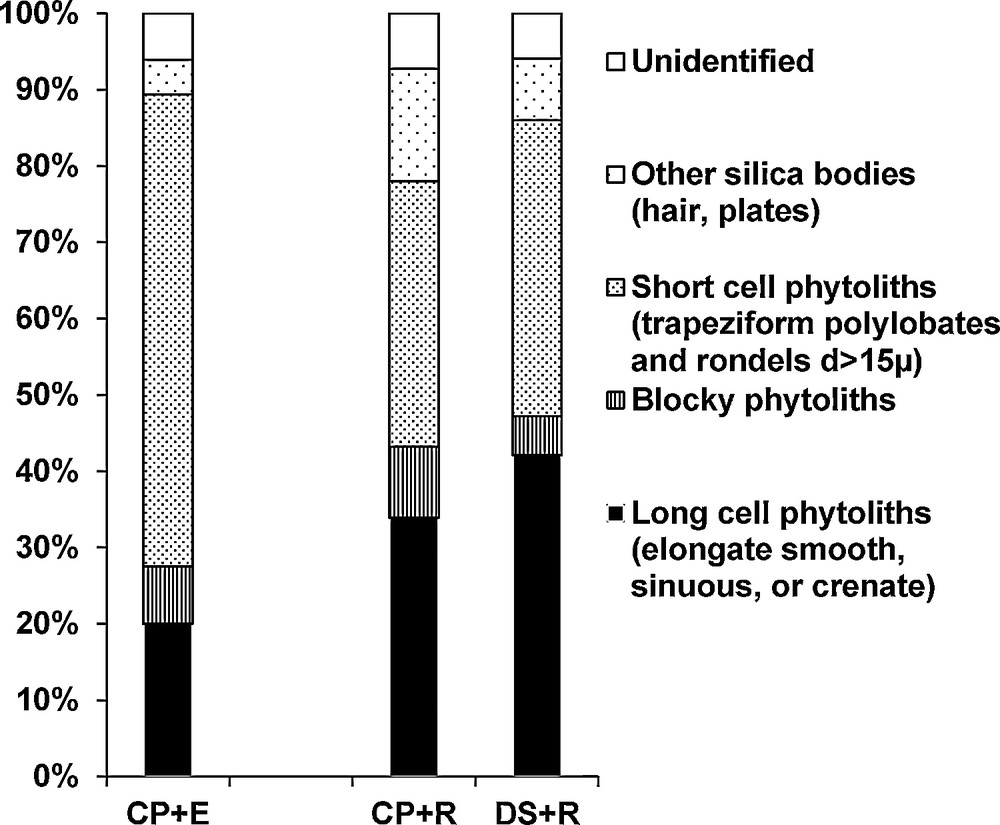
Relative abundance (in %) of phytolith morphotypes in topsoil samples that underwent straw restitution at Arvalis experimental station, Boigneville, France. CP, DS, E and R = see Fig. 2.
Abondance relative (en %) des types morphologiques de phytolithes dans les horizons de surface de l’expérience de restitution de paille à Boigneville, station expérimentale d’Arvalis, France. CP, DS, E et R = voir Fig. 2.
Therefore, straw exportation may affect the ASi pools even after a decade. The present results are also in agreement with the experiments showing the progressive increase of soluble Si (available to plants) and Si concentrations in rice crops following incorporation of rice straw into soil (Datnoff et al., 2001).
5 Summary and recommendation
Evidence from the literature and original data suggests that crops modify the silicon biogeochemical cycle through both accelerated Si uptake due to crop plants being more efficient than natural vegetation at taking up nutrients and other elements, and Si export through total or partial shoots removal from the field. The resulting trend is a decrease in long term phytoavailable (less phytoliths) and short term phytoavailable Si (soluble) leading to lower Si concentrations in plants and probable lower Si exports out of the soil. The consequences are: (a) putative changes in the various Si pools in soil and their respective contribution to Si cycle (Carey and Fulweiler, 2011; Struyf et al., 2010a); (b) a possible impact on crop yield and plant health (Savant et al., 1997). The extent of these changes is not enough documented, neither is the impact of Si soil depletion on the long term. More case studies are needed to determine the contribution of other factors that can lead to phytoliths depletion in cultivated land: increasing of soil erosion (Conley et al., 2008) and phytoliths mobility downwards the soil profile (Fishkis et al., 2010).
There is thus a need for characterizing the various Si pools in soil including their dissolution kinetics and their relative contribution to amorphous silicon and phytoavailable silicon. The role of pH needs to be better assessed for constraining the evolution of phytoliths storage in the biogeochemical cycle. It has been recently suggested that the application of nitrogen fertilizers may enhance silicate dissolution because of oxidation of NO3− into NH4+ producing proton (Fortner et al., 2012). Lower pH may increase the delivery of DSi from various minerals into soil solutions to counterbalance the phytoliths loss. Indeed, Makabe et al. (2009) found that available Si (phosphate buffer extraction at pH 6.9) in paddy fields in Japan was related to mineralogy and positively correlated to the amount of the clay fraction. The relative contribution of clays minerals, phytoliths and other amorphous Si forms to the phytoavailable Si pool needs to be clarified. This would allow using Si as a putative tracer of soil element depletion in European soils where silicon losses are not balanced by fertilisers inputs.
Acknowledgements
This work has been financed by EC2CO, a French national scientific program of CNRS (INSU).