1 Introduction
Silicon (Si) was born in the universe by fusion between atoms of oxygen (O). It is one of the most abundant elements of the universe, the second most abundant for planet Earth. Si atoms form bonds with O atoms to create silica (SiO2), which may be either crystalline or amorphous, with biogenic forms of silica and volcanic glasses being amorphous and minerals such as quartz being crystalline. Similar silica structures form the basis of rock-forming silicate minerals (such as kaolinite and other clays, micas, olivines, pyroxenes, feldspars, and plagioclases). Weathering (dissolution) of silicate rocks and minerals at low or high temperatures generates silicic acid (H4SiO4 or Si(OH)4), so-called dissolved silica (herein abbreviated as DSi). Discharge of rivers and of submarine groundwater into the coastal ocean, hydrothermal inputs into deep waters, dissolution of siliceous material transported from the continents to the continental margins and that of air-borne suspended materials in surface waters are pathways for DSi input into the ocean. In surface waters, DSi is taken up by diatoms to build their frustules of amorphous biogenic silica (bSiO2). Removal of DSi from the ocean corresponds mostly to the burial of biogenic silica, as opal, in abyssal and coastal sediments, although a minor contribution from siliceous sponges might also be involved. A revised budget of Si in the world ocean (Fig. 1) has been published by Tréguer and De La Rocha (2013). Given the large uncertainties in the fluxes of DSi and of bSiO2 (Tréguer and De La Rocha, 2013), the question of the steady state of the Si cycle in the modern ocean remains an open question.
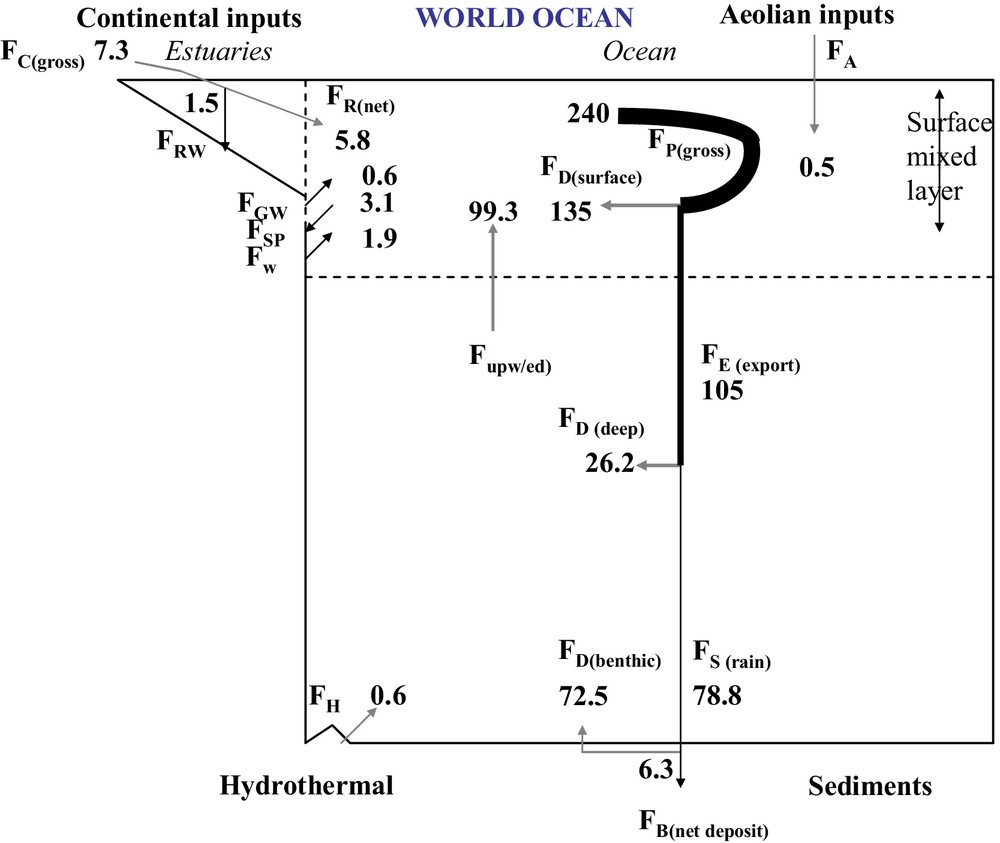
Biogeochemical cycle of silicon in the world ocean at steady state (a possible balance that is in reasonable agreement with the individual range of each flux, F). Gray arrows represent fluxes of silicic acid – dissolved silica, DSi – and black arrows represent fluxes of particulate biogenic silica; the vertical dotted line represents the limit between the estuaries and the ocean; the horizontal dotted line represent the limit between the surface well-mixed reservoir and the deep ocean. All fluxes (F) are in 1012 or teramoles of silicon per year.
For details, refer to Tréguer and De La Rocha (2013).
The key role played by the Southern Ocean in the control of the world ocean silica cycle was identified long ago (e.g., Anderson et al., 2002; DeMaster, 1981, 2002; Nelson et al., 1995; Pondaven et al., 2000; Tréguer et al., 1995). On the one hand, at subsurface and intermediate depths, Sub-Antarctic Mode Water and Antarctic Intermediate Water export huge amounts of DSi that remains unused by siliceous phytoplankton in Southern Ocean surface waters (Fig. 2). In the abyss, DSi-rich Antarctic Bottom Water also exports DSi into the deep areas of the Atlantic, Indian, and Pacific basins (Anderson et al., 2002). On the other hand, the large opal belt of opaline sediments that girds the Antarctic, roughly underlying the Polar Frontal Zone, has been cored for several decades (e.g., DeMaster, 1981, 2002). The impressive abundance of biogenic silica in the opal belt sediments makes the Southern Ocean one of the most important silica sinks in the world ocean (DeMaster, 2002; Pondaven et al., 2000). So far however, the Si status of the Southern Ocean is unclear: is the Southern Ocean a net sink or a net source of DSi for the rest of the world ocean? This is the question addressed in this study.
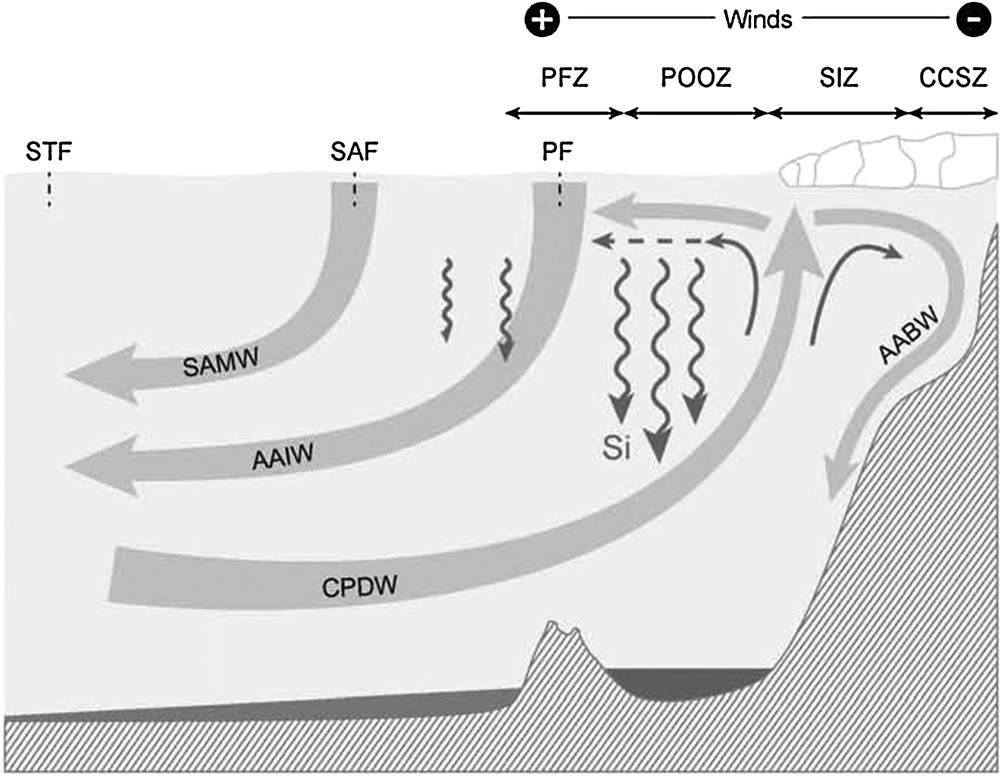
The Southern Ocean: schematic vertical circulation of water masses, frontal systems, major ecosystems and vertical transport of biogenic silica. Fronts: PF: polar front; SAF: Sub-Antarctic Front; STF: Sub-Tropical Front; note that we use to call “Antarctic Ocean” the part of the SO which comprises the polar front zone (PFZ), the permanently open ocean zone (POOZ), the seasonal ice zone (SIZ) and the coastal and continental shelf zone (CCSZ). Through the upwelling of the Circumpolar Deep Water (CPDW) at the Antarctic divergence, the surface waters are enriched in silicic acid. This favors the growth of diatoms and intense biogenic silica production, which triggers abundant export of biogenic silica to deep waters ultimately depositing opal on abyssal sediments (mostly south of the PF). The silicic acid unused in surface waters is exported to the rest of the world ocean through the Sub-Antarctic Mode Water (SAMW), the Antarctic Intermediate Water (AAIW), and the Antarctic Bottom Water (AABW).
Preliminary note: we use herein the distinction introduced by Tréguer and Jacques (1992) between the Southern Ocean and the Antarctic Ocean. According to these authors, the “Antarctic Ocean” (usually located south of 50°S) comprises four major ecosystems (cf. Fig. 2): the polar front zone (PFZ, 3 millions of km2), the permanently open ocean zone (POOZ, 14 Mkm2), the seasonal ice zone (SIZ, 16 Mkm2) and the coastal and continental shelf zone (CCSZ, 0.9 Mkm2).
2 The Si cycle in the Southern Ocean
2.1 The Input Fluxes
No river or submarine groundwater discharge to the ocean can exist from a frozen continent. So, a priori FRnet and FGW are null for the Antarctic.
In their silica balance of the world ocean, Tréguer and De La Rocha (2013) did not consider the potential DSi input to the ocean due to the melting of the Antarctic and the Greenland Ice Sheets (FISMW). This DSi flux is fed by the discharge of subglacial meltwaters from the ice sheets, the basal melting of the ice shelves, and the melting of icebergs. So far, compared to the world river discharge (39,080 km3·yr−1), fluxes of meltwater from polar ice sheets are modest. However, it has been shown that they can be a significant source of Fe to the oceans (e.g., Hawkings et al., 2014; Wadham et al., 2013). Some of these fluxes might be DSi-rich. So, do they matter for the global silica balance?
1. Contribution of the subglacial meltwaters of the ice sheets: liquid water is present at the beds of polar ice sheets, and flows via a diverse suite of hydrological environments, including rivers, groundwaters, and subglacial lakes. The latter are particularly well developed in Antarctica (Wright and Siegert, 2012). It results from geothermal flux, ice deformation and ice bed friction (Flament et al., 2014), so that 55% of the grounded part of the Antarctic ice sheet is at the pressure melting point (Pattyn, 2010). Only the basal meltwater produced beneath the ice streams around the Antarctic periphery is likely to discharge into the ocean (Pattyn, 2010), the potential flux being approximately 65 Gt·yr−1 or about 65 km3·yr−1 (Pattyn, 2010). This is about six times lower than that for Greenland, but the solute concentrations in the Antarctic meltwaters are expected to be much higher (at least one order of magnitude higher) because of the very long residence time of the Antarctic meltwaters in subglacial environments compared to Greenland (Wadham et al., 2013). Subglacial outburst events in Greenland, which are believed to reflect the rapid discharge of meltwaters stored at the ice sheet bed over winter, might represent the most relevant analogue for Antarctic subglacial meltwaters. DSi concentrations ranging between 0.76 and 41.4 μM have been measured in subglacial outburst meltwaters in Greenland, the mean being 9.55 μM (J. Hawkings, pers. comm.). So, a preliminary estimate of the Greenland meltwater maximum discharge could be (390·109 m3·yr−1) × 41.4 mmol·m−3 = 0.016 TmolSi·yr−1. Values of DSi concentrations in the Antarctic subglacial meltwaters have not been published so far; however Wadham et al. (2013) gave estimates ranging between 6 and 600 μM. So, the maximum DSi flux of glacial meltwater to the Southern Ocean should be (65 109 m3·yr−1) × 600 mmol·m−3 = 0.039 TmolSi·yr−1.
2. Contribution of the ice shelves and of the melting of icebergs: ice shelves are well extended in Antarctica compared to Greenland, where the ice sheet meets the sea in fjords: they cover an area of > 1.5 Mkm2, fringing 75% of the Antarctic coastline (Rignot et al., 2013). The ice shelves lose mass through topside sublimation, wind drift, iceberg calving and basal melting (Rignot et al., 2013). The ice-shelf basal melting is the largest ablation process in Antarctica (Rignot et al., 2013). The basalt melt flux and the calving flux are estimated at 1325 (± 235) Gt·yr−1 and 1089 (± 139) Gt·yr−1, respectively (Rignot et al., 2013). So, the total ice-shelf meltwater production (including the ultimate melting of the icebergs) for Antarctica is 2414 (± 374) Gt·yr−1. The iceberg calving flux is a major component of the total mass balance of the Greenland Ice Sheet (Bamber et al., 2012; Bigg et al., 2014); since 2006 high summer melt rates have increased Greenland Ice Sheet mass loss, which is estimated at about 270 (± 40) Gt·yr−1 (Van den Broeke et al., 2009). Ice shelves and free-drifting icebergs contain terrigenous debris at their base, including ferrihydrite, which disperses in seawater during melting and eventually dissolves (Shannon et al., 2013; Smith, 2011). As a result, free-drifting icebergs have been considered as hot spots of chemical and biological enrichments (Smith et al., 2007).
Does meltwater from basal melting of the ice shelves and/or from icebergs contain significant amounts of DSi? A priori, pure ice melt is expected to contain negligible amounts of DSi. However, in the Hodgson lake water, one of the former Antarctic subglacial lake that emerged from under the ice but remains perennially ice-covered, Hodgson et al. (2009) measured DSi concentrations ranging between 3.7 and 20.5 μM (mean: 13.4 μM), giving indication for assessing the DSi concentrations in glacial ice. As regards meltwaters resulting from the basal melting of the ice shelves and from the melting of icebergs, few attempts have been made to determine their DSi impact. Recently, in the strong outflow from under the western end of the Dotson ice-shelf (Antarctica), no measurable DSi anomaly was observed (R. Sherrell, pers. comm.). In April 1987, during the Scotia Sea Apsara III cruise (Tréguer et al., 1990), surface seawater was sampled both in the immediate wake of a large tabular iceberg and in waters remote from the berg. Although continuous salinity measurements show a slight decrease in salinity (< 0.1 pss) in the immediate wake of the iceberg no significant difference in DSi concentration was observed (P. Tréguer, unpublished data). Vernet et al. (2011) studying the impact of free-drifting icebergs in the NW Weddell Sea, sampled surface waters close and far from the icebergs. Close to the bergs they measured a negative salinity anomaly (< 0.1 pss), an increase in nitrate concentration (a few tenths of μM), but no significant difference for DSi. However, strong dilution of the meltwater by the warm modified Circumpolar Deep Water for the Dotson ice-shelf, or by the Antarctic surface water for the Scotia Sea and the Weddell Sea, might have hidden the DSi impact of meltwater.
So, FISMW is the sum of:
- • the DSi flux of the subglacial meltwater discharge (ice sheet);
- • the DSi flux of meltwater derived via basal melting of ice shelves;
- • the DSi flux of the meltwater of the icebergs.
Maximizing FISMW for the Antarctic would give: FISMW = (65·109 × 600·10−3 + 1560·109 × 20.5 10−3 + 1228·109 × 20.5·10−3) = 0.097 TmolSi·yr−1. For Greenland, neglecting the contribution of the basal melting of floating ice tongues, the maximum value for FISMW is (390·109 × 41.4·10−3 + 310·109 × 20.5·10−3) = 0.022 TmolSi·yr−1. Thus, compared to the other DSi input fluxes (Fig. 1 and Tréguer and De La Rocha, 2013), we herein consider that FISMW can be neglected, both for the Antarctic and for Greenland (Table 2).
Silica cycle in the Southern Ocean and in the world ocean silica: Si sources and sinks, fluxes in tera (1012) mol of Si·per year.
Southern Ocean | Rest of the word ocean | Total | |
DSi inputs | |||
Rivers DSi (FRnet) | 0 | +5.8 ± 2.5 | +5.8 ± 2.5 |
Groundwater (FGW) | 0 | +0.6 ± 0.6 | +0.6 ± 0.6 |
Aeolian (FA) | < 0.005 | +0.5 ± 0.5 | +0.5 ± 0.5 |
Hydrothermal (FH) | < 0.04 | +0.6 ± 0.4 | +0.6 ± 0.4 |
Margins (FW) | ? | +1.9 ± 0.7 | +1.9 ± 0.7 |
Meltwater (ice sheets) (FISMW) | < 0.1 | < 0.02 | < 0.1 |
Total inputs | 0 | +9.4 ± 4.7 | +9.4 ± 4.7 |
bSiO 2 outputs | |||
Opal burial (FB(net deposit)) | –2.0 ± 1.2 | –4.3 ± 2.4 | –6.3 ± 3.6 |
Sponges (FSP) | ? | –3.6 ± 3.7 | –3.6 ± 3.7 |
Total outputs | –2.0 ± 1.2 | –7.9 ± 6.1 | –9.9 ± 7.3 |
bSiO2 production (FP(gross)) | 80 ± 18 | 160 ± 22 | 240 ± 40 |
Opal preservation (%) in sediments | 2.5 ± 0.07 | 2.7 ± 0.11 | 2.6 ± 0.18 |
Dry deposition of air-borne particulate material (mostly lithogenic) is especially low over the Southern Ocean in the present times (e.g., Mahowald et al., 2005; Tegen and Kohfled, 2006). With an average of 0.2 g·m−2·yr−1, the total dust deposition at the surface Southern Ocean (33.9 Mkm2) is 6.8 Mt·yr−1, which is between 0.35 and 0.7% of the world ocean total deposition. Only a small fraction (1–10%) of the deposited particulate lithogenic silica dissolves in seawater (Tegen and Kohfled, 2006). Thus, the corresponding DSi annual flux, FA should be negligible; although no accurate determination is available our best guess value is that it should stand < 1% of the global total (0.5 ± 0.5 TmolSi·yr−1, Tréguer and De La Rocha, 2013). So, the value of the Southern Ocean FA should be < 0.005 (± 0.005) TmolSi·yr−1 (Table 2).
Hydrothermal activity has been reported in the Southern Ocean > 50°S (e.g. in the Scotia Sea or in the Bransfield Strait, German et al., 2000; Klinkhammer et al., 2001; Rogers et al., 2012). However, so far no data allows estimating FH, the DSi annual flux generated by deep-sea high-temperature activity at mid-ocean ridges (axial component of the flux) and by low-temperature activity at ridge flanks (off-axis component). Tivey (2007) localized four sites of hydrothermal venting in the Southern Ocean (> 50°S) among the 61 active sites reported for the world ocean. This represents (4/61) = 6% of the total number of sites. Given that the global hydrothermal flux of DSi (0.6 ± 0.4 TmolSi·yr−1, Table 2) includes not only axis systems but also ridge flank hydrothermal systems (details in Tréguer and De La Rocha, 2013), our best guess for a tentative value of the DSi hydrothermal flux of the Southern Ocean is < 6% of the global total. So, the Southern Ocean FH should be < 0.04 (± 0.02) TmolSi·yr−1 (Table 2).
No data is available for the Southern Ocean to estimate FW, the DSi flux that results from the low-temperature dissolution of terrigenous material transferred from the Antarctic continent (for instance by the icebergs) to the continental margin, and from the sea-floor basalt. However, because deep and bottom waters of the Southern Ocean are DSi-rich, we expect saturation of the fluid with respect to numerous silicate minerals, so that the value of FW should be negligible for the Southern Ocean compared to the rest of the world ocean (Table 2).
2.2 The biogeochemical fluxes
Silica production: Pondaven et al. (2000) calculated the biogenic silica annual production for the PFZ, the POOZ and the SIZ of the Indian sector of the Southern Ocean. Extrapolating to the whole Antarctic Ocean gives an annual production of: 7.3 (±1.2), 41.8 (±7.6), 25.9 (±9.3) TmolSi·yr−1 for the PFZ, the POOZ and the SIZ, respectively. The grand total is 80 (±18) TmolSi·yr−1. Note that this estimate of the FP(gross) does not include the production of the continental and shelf zone CCSZ (for which no annual estimate is available).
Losses due to silica dissolution in the photic zone: A part of the gross production (FP(gross) or P) is recycled in the surface layer via the dissolution (FD(surface) or D) of the biogenic silica. As measured from time-series (Table 1 in Tréguer and De La Rocha, 2013) the D:P mean ratio in the Southern Ocean ranges between 0.16 in the Sub-Antarctic zone (SAZ) and 0.74 in the CCSZ; D/P averages at 0.26 in the PFZ and 0.53 in the SIZ. From the surface of these different zones (Tréguer and Jacques, 1992, also see above) the ratio-weighted average of D:P is 0.50, not much different from 0.56, the mean value adopted by Tréguer and De La Rocha (2013) for the world ocean. Given the lack of data in the POOZ, which represents 47% of the total surface of the Antarctic Ocean, the D:P mean ratio herein adopted is 0.56 (Table 2, Fig. 3), i.e. identical to that of the world ocean.
Making explicit the DSi and biogenic fluxes in the surface and deep reservoir of the Southern Ocean.
DSi flux | |
F C(gross) | The total river gross discharge to the world ocean (for details see Tréguer and De La Rocha, 2013) |
F R(net) | The total river net discharge to the world ocean |
F A | The dissolution of aeolian-transported siliceous dusts |
F GW | The submarine groundwater discharge |
F W | The dissolution of siliceous material transported from land on the continental margins |
F H | The hydrothermal activity of the oceanic ridges (axis + off axis) |
F ISMW | The ice-shelf melt water flux (subglacial melt water + basal melting of ice shelves + melting of icebergs) |
F D(surface) | The recycling of Si by dissolution of the biogenic silica in the surface reservoir |
F D(deep) | The part of the export flux that dissolves in the deep reservoir |
F D(benthic) | The flux at the sediment–water interface |
F upw/ed | The transfer from the deep to the surface reservoir by upwelling or eddy diffusion |
bSiO 2 flux | |
F Pgross | The production of biogenic silica due to diatoms |
F E(export) | The export flux of biogenic silica to the deep reservoir |
F S(rain) | The part of the export flux that reaches the sediment – water interface |
F B(net deposit) | The long-term accumulation of biogenic opal in coastal and abyssal sediments |
F SP | The net removal due to siliceous sponges |
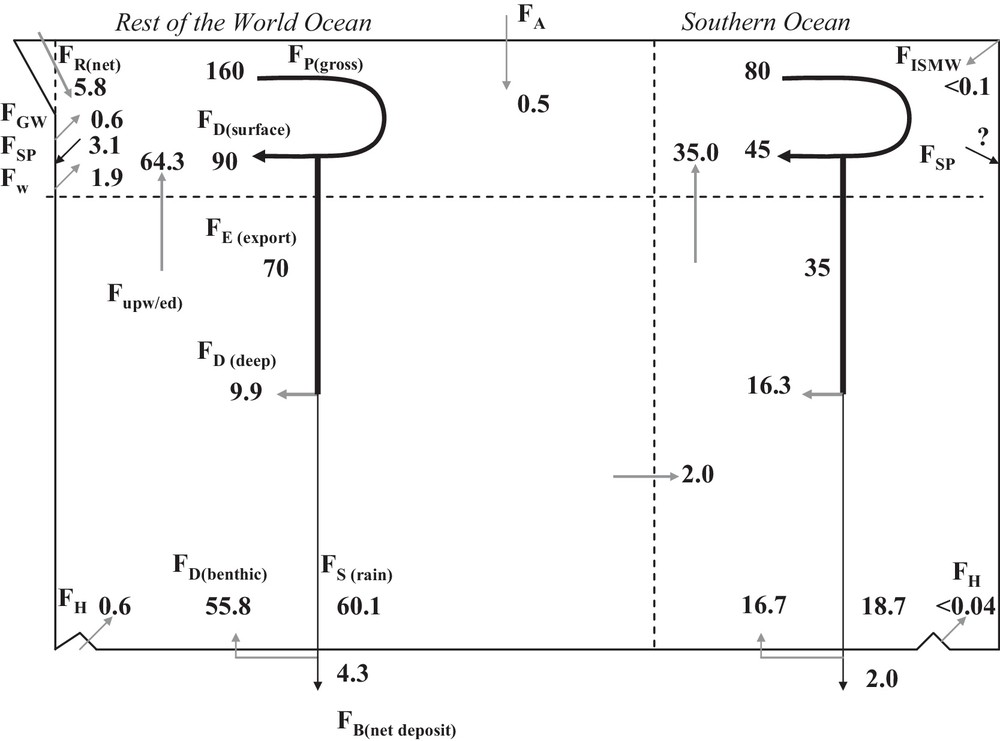
Biogeochemical cycle of silicon in the world ocean at steady state, with distinction of the Southern Ocean and of the rest of the world ocean (a possible balance that is in reasonable agreement with the individual range of each flux, F). Gray arrows represent fluxes of silicic acid (DSi), and black arrows represent fluxes of particulate biogenic silica (bSiO2); the horizontal dotted line represent the limit between the surface well-mixed reservoir and the deep ocean; the vertical dotted line on the left represents the limit between the estuaries and the ocean; the vertical dotted line on the right represents the limit between the Southern Ocean (Polar Front) and the rest of the world ocean; all fluxes (F) are in teramoles (1012) of silicon per year (TmolSi·yr−1). Abbreviations: see Table 1.
Si recycling at the water-sediment interface: the values of the diffusive flux of DSi (FD(benthic)), calculated from measurement of gradients in sediment pore waters or by using benthic chambers are sensitive to methodology (Ragueneau et al., 2000; Tréguer and De La Rocha, 2013). So far, only few data are available for the Antarctic continental margins and the abyssal sediments. The diffusive flux ranges between 7.7 (pore water gradients) and 37.3 TmolSi·yr−1 (benthic chambers). For Fig. 3, a tentative flux of 16.7 TmolSi·yr−1 has been taken, in reasonable agreement with the range of FD(benthic). Note that such FD(benthic) flux corresponds to a flux of opal to the sea-floor (FS(rain)) of 18.7 TmolSi·yr−1, which is about (18.7/80) = 23% of the gross production in surface waters. Interestingly (because the estimates are independent), this appears to be quite consistent with estimates for the Pacific sector calculated by Nelson et al. (2002).
2.3 The output fluxes
Opal accumulation in sediments vs. biogenic silica production in surface waters: the nutrient concentrations of Southern Ocean waters are among the richest of the world ocean. As regards the silicic acid concentration, the Southern Ocean just stands after the North Pacific Ocean, whose DSi-richness is explained by intensive recycling of Si in deep and bottom waters at the end of the northward journey of the global “conveyor belt” (Rahmstorf, 2003). The DSi-rich Antarctic surface waters support high diatom abundance and biogenic silica production, which in turn supports high export production to the ocean interior, and ultimately high opal accumulation rates in sediments (DeMaster, 1981). However and paradoxically, the Antarctic surface waters are renowned for their suboptimal primary production (e.g., Pondaven et al., 2000). The growth of Antarctic phytoplankton (including diatoms) is severely limited by a lack of dissolved iron in surface waters (e.g., Sarthou et al., 2005). Until the end of the 20th century, on the one hand biogenic silica production associated with the primary production of diatoms in surface waters was underevaluated, while on the other hand the burial rate of opal in abyssal sediments was overestimated, creating the so-called Antarctic Ocean “opal paradox” (Pondaven et al., 2000). Consistent with thermodynamics, dissolution of biogenic silica in the cold surface and deep waters of the Southern Ocean is especially low. Nelson et al. (1995) postulated that the preservation of opal (estimated by the ratio of the rate of the long-term burial of opal in sediments to that of biogenic silica production in surface waters) was more efficient in the Southern Ocean than elsewhere. However, using new techniques (including 230Th-normalized accumulation rates to correct from sediment focusing, cf. Pondaven et al., 2000), it was demonstrated that the opal preservation ratio is less than 3%, i.e. as low in Antarctic sediments as it is for the rest of the world ocean, something which was confirmed by DeMaster (2002). Tréguer and De La Rocha (2013) calculate FB(net deposit) of the Antarctic Ocean, taking only into account of 230Th-normalized opal accumulation rates (for details about the method, see Pondaven et al., 2000). From 93 data points acquired in the Atlantic, Indian and Pacific sectors of the Southern Ocean, the total long-term burial rate of opal, FB(net deposit), is estimated at 2.0 ± 1.2 TmolSi·yr−1, the majority of this opal accumulation occurring south of the PF (Fig. 2). Note that this estimate of FB(net deposit) does not include the opal burial occurring in CCSZ, for which no 230Th-normalized opal accumulation rates are available.
Thus, the preservation ratio of opal in the Antarctic abyssal sediments (excluding the CCSZ) is (2.0 (±1.2)/80 (±18) = ) 2.5% (± 0.07%), which is not significantly different from the preservation ratio of the world ocean: 2.7% (± 0.18%), see Table 2.
Siliceous sponges as a silica sink: siliceous sponges are biogenic silica producers and as such are comparable to diatoms. However, herein they are taken as contributors to the ocean silica sink in coastal and deep environment (Maldonado et al., 2005; Tréguer and De La Rocha, 2013). This is, first, because compared to short-lived diatoms, sponges usually live from years to decades or even centuries, and, second, because the siliceous spicules of sponges resist dissolution (Maldonado et al., 2005). Sponges represent a major component of the Antarctic zoobenthos and abundant colonies of siliceous sponges have been reported in the many embayments of the Antarctic coastal zone (e.g., in the Terra Nova bay, Ross Sea, Cattaneo-Vietti et al., 2000). For the Antarctic Peninsula, Fillinger et al. (2013) describe rich benthic communities dominated by glass sponges, whose growth is supported by a patchy primary production during seasonal sea-ice melting; they show that rapid expansion of glass sponges follows climate-induced ice-shelf collapse. In the McMurdo Sound, Ross Sea, Dayton et al. (1974) and Dayton (1979), followed, for a period of ten years, colonies of siliceous sponges growing at 40 to 60 m depth in a coastal area of 22,000 m2. Reanalysing Dayton's data for sponge growth and weight to wet weight ratios of the demosponge Momaxinella balfouriensis, Maldonado et al. (2005) calculated an estimate of 8.37 molSi·m−2·yr−1 for the mean bSiO2 production rate. Interestingly, this is 60% of the mean silica production of diatoms in the corresponding water column. Unfortunately, no data is available regarding the dissolution rate of Antarctic siliceous sponges. This lack of information prevents us from giving a realistic estimate for the FSP of the Antarctic Ocean.
3 The Southern Ocean vs. the rest of the world ocean
Fig. 3 shows a tentative chart of Si fluxes in the Southern Ocean compared to the rest of the World Ocean.
This portrayal of the silica cycle of the Southern Ocean suggests that:
- • although silica productive, the Southern Ocean contributes only 33% of both the total gross production and export production of the world ocean. Using an optimized ocean model and a Green function to describe the matter fluxes, Holzer et al. (2014) calculate an export biogenic silica production as high as 106 (±17) TmolSi·yr−1, which is 70% of its world total. Based on measured biogenic silica production and D:P ratios, our estimate of the Southern Ocean export flux out of the photic zone ranges between 27 and 43 TmolSi·yr−1. Thus, Holzer et al.’s model might underestimate the dissolution of biogenic silica in the photic zone;
- • traditionally, the Southern Ocean is considered as a major exporter of nutrients to the rest of the world ocean, first at the subsurface and at intermediate depths through the Sub-Antarctic Mode Water and the Antarctic Intermediate Water pathways; the Antarctic Bottom Water, mainly formed in the Weddell and Ross Sea (although smaller embayments like the Amery Sea might contribute, Smith and Tréguer, 1994), also exports DSi to deeper waters of the Atlantic, Indian and Pacific oceans. Sarmiento et al. (2004) showed that three quarters of the primary production in surface waters north of 30°S is supported by inputs from the Southern Ocean, so that the Southern Ocean can be envisioned as a strong DSi exporter. However, we herein show (Fig. 3) that, because there is no specific pathway for delivering DSi input from the Antarctic continent, the Southern Ocean is actually a net importer of DSi. At the steady state, through the Circumpolar Deep Water, the Southern Ocean imports 2.0 (± 1.2) TmolSi·yr−1 to compensate for the biogenic silica sink in sediments.
4 Conclusions
The Southern Ocean is like a hub that charges taxes to the DSi that wants to pass through. To our knowledge this is the first study taking into consideration all the fluxes that control the silica cycle of the Southern Ocean. There is still much unknown and uncertainty as regards the input and output fluxes, as well as the cycling of Si in the surface layer, in the deep waters, and at the water-sediment interface.
Three efforts are recommended for the future.
- • first, to constrain the input flux of DSi, we need to better know: (1) the range and variability of DSi concentrations in the melting waters of the ice sheets and in the basalt melting of the ice shelves, a pathway of DSi input to the Southern Ocean which should increase with climate warming (e.g., Mulvaney et al., 2012), and (2) the flux of dissolution of the siliceous lithogenic material that is deposited on the continental margins by the icebergs;
- • second, as regards the long-term opal accumulation rates for the coastal zone and the continental margins, while data are already available for the Ross Sea, there is still much to do for the rest of the Southern Ocean;
- • last but not least, the DSi-rich waters of the Southern Ocean appear to be a favourable realm for siliceous sponges. So far however, we do not know much about the DSi and bSiO2 flux controlled by siliceous sponges in Antarctic waters.
Acknowledgements
The author thanks Christina De La Rocha (IUEM-UBO), Gregory de Souza (Princeton University), Thomas Flament (LEGOS), Jon Hawkings (University of Bristol), and Rob Sherrell (Rutgers University) for fruitful exchanges. Special thanks are due to David M. Nelson (IUEM-UBO), for his help in finalising and editing the manuscript, to Jemma Wadham (University of Bristol), for discussion on the impact of the ice sheet melting on the polar oceans, and to Amaëlle Landais and Vincent Courtillot, for their comments and questions on a first version of this manuscript.