1 Introduction
The boreal zone of the Russian Arctic and glaciered systems of Greenland and Alaska are systems that are currently experiencing rapid environmental change associated with climate change. The observed warming in the Arctic is much greater than the global average (IPCC, 2007) and on-going permafrost thaw is considered to induce large perturbations on the global water discharge and organic carbon inventory in Arctic rivers (Dittmar and Kattner, 2003; Holmes et al., 2012) as well as the flux and speciation of trace elements input into the Arctic Ocean (Pokrovsky and Schott, 2002; Pokrovsky et al., 2010, 2012). The Arctic Ocean receives about 10% of the global river discharge, yet it has the highest input of continental freshwater per basin surface area compared to all other oceans in the world. In addition, the three largest Arctic rivers, the Yenisey, Lena, and Ob’, are each comparable in watershed area and annual discharge to the Mississippi River (Holmes et al., 2012).
Riverine iron (Fe) plays a critical role in regulating the concentration and bioavailability for a variety of chemical elements in aquatic ecosystems, including nutrients and pollutants. In general, the behavior of Fe and its partitioning between dissolved, colloidal, and suspended sediment loads is controlled by local hydrogeochemical and biogeochemical environments that are themselves likely to be affected by climate change (Allard et al., 2004; Schroth et al., 2009). Likewise, glacial weathering has been recently recognized as a primary source of Fe and other nutrients (phosphate, dissolved organic matter) to the highly productive coastal ecosystems of the Gulf of Alaska (Crusius et al., 2011; Schroth et al., 2011; Hood and Scott, 2008; Schroth et al., 2014) and enhanced iron input has been observed during high run-off periods of snowmelt in spring and glacial melt in the summer. Understanding the mechanisms and external forcing of Fe delivery into the Arctic Ocean therefore requires:
- • time-series-based analyses of Arctic and sub-Arctic river biogeochemistry to assess climatic and seasonnally-driven variations;
- • assessment of the speciation and mobility of Fe in high-latitude watershed;
- • determination of the potential impact of glacier or permafrost thaw on the speciation, timing and provenance of Fe input to the high-lattitude oceans.
A growing number of studies have reported Fe isotope compositions of bulk rivers, as well as particulate, dissolved and colloids Fe pools in rivers (Bergquist and Boyle, 2006; Chen et al., 2014; Escoube et al., 2009; Fantle and DePaolo, 2004; Ilina et al., 2013; Ingri et al., 2006; Pinheiro et al., 2013, 2014; Poitrasson et al., 2014; Schroth et al., 2011). The results showed significant variability in Fe isotopes, which has been attributed to a range of processes and parameters, including hydrology, climate, and anthropogenic influences, as well as bedrock geology, topography, and soil–plant interactions. To date, only two studies have reported the isotope composition of dissolved Fe in Arctic/sub-Arctic environments (i.e. referred to as δ56FeDFe, with DFe for dissolved Fe < 0.45 or < 0.22 μm) that yielded one of the largest range observed in river systems, between–1.2 and 1.8 ‰ (Ilina et al., 2013; Schroth et al., 2011). The lightest δ56FeDFe values were reported for organic-rich rivers and stream-draining areas, with the largest vegetal cover in Alaska (Schroth et al., 2011), while the heaviest values were measured in colloidal and dissolved fractions of boreal and temperate organic-rich rivers in Karelia (Ilina et al., 2013).
Here, we investigate Fe isotope systematics in northern European and Siberian rivers (Fig. 1), including:
- • a time-series of δ56FeDFe of two of the largest rivers draining Arctic watersheds (the Ob’ and Lena) focusing on the peak flow that provides a first-order assessment of the annual Fe budgets;
- • δ56Fe values of colloidal and suspended pools (ranging from 1 kDa to 1.2 μm) of smaller northern European rivers, including the Severnaya Dvina River to assess the influence of Fe-rich colloids on the Fe isotopic composition of freshwater sources in the Arctic.
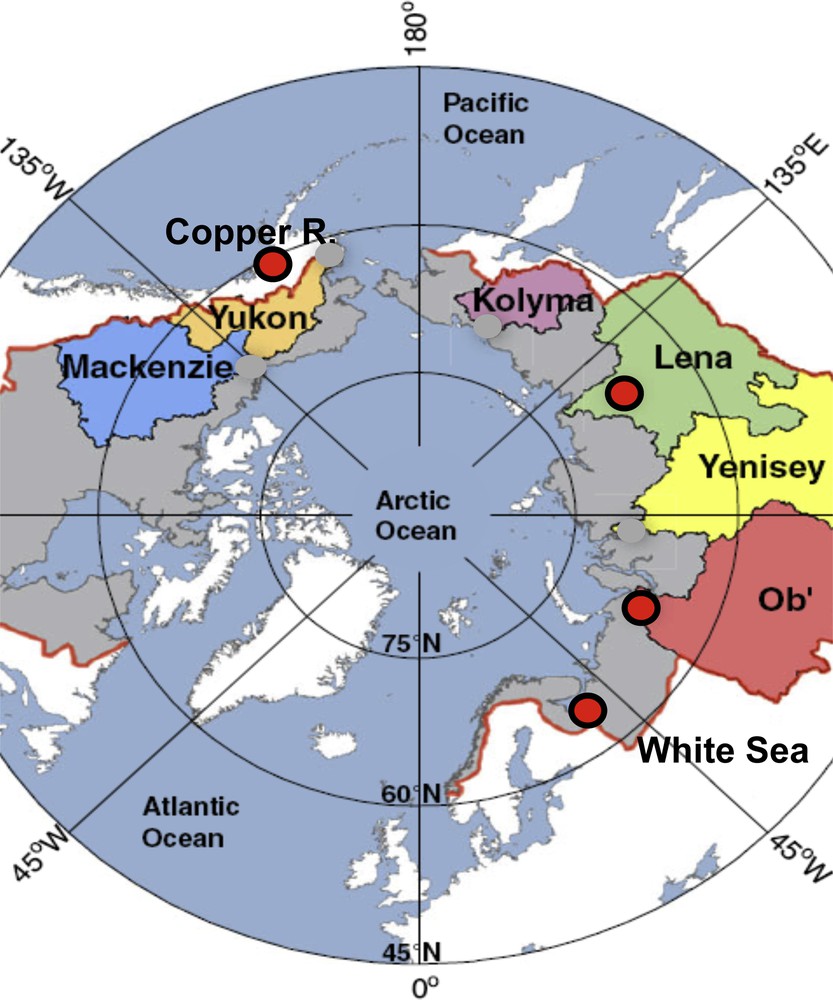
(Color online). Map showing the watersheds of the major rivers discharging in the Arctic Ocean and adapted from Holmes et al. (2012). Red dots show sampling locations of the Ob’ and Lena, Copper River and its tributaries, as well as rivers draining into the White Sea. The Ob’, Lena and Severnaya Dvina Rivers contribute respectively 18%, 25% and 4.6% of the total riverine water flux in the Arctic Ocean.
We further compare the results with our previously reported Fe isotope composition of Alaskan rivers (Schroth et al., 2011) to determine how Fe isotope signatures may vary among high-latitude watersheds. Without such characterization of the present state of the system, future changes in the response of these river systems to global change cannot be properly evaluated.
2 Materials
Field sampling of the Ob’ and Lena have been performed by the Arctic Great Rivers Observatory during the 2007 base-flow-to-high-flow transition, as reported by Holmes et al. (2012). The sampling sites were located the nearest to the river mouths at Salekhard for Ob’ and Zhigansk for Lena. With a discharge of 427 km3/year and 588 km3/year, respectively, the Ob’ and Lena represent 18 and 25% of riverine freshwater inputs to the Arctic Ocean (Holmes et al., 2012).
Rivers draining into the White Sea (between latitudes 67°N and 63°N and longitudes 30°E and 36°E) were sampled in the boreal and sub-Arctic region of European Russia. This region typically experiences a very large range of temperature varying from –50 °C to 30 °C between winter and summer. The mean annual river discharge in the White Sea is 231 km3/yr, out of which 47% correspond to the Severnaya Dvina watershed (Pokrovsky et al., 2010). The sampled sites are on an unpopulated area and represent a diverse collection of geochemical environments with bedrock lithologies ranging from granites, basalts, ultramafic rocks, and carbonate-rich sediments (Table S1, Supplementary material) and hydrological settings (soil depression, river, bog and meltwater). Based on the geographic location, we divided the studied area into three zones:
- • Yukova watershed, including the Yukova, Ladreka and Ruiga Rivers and stagnant waters;
- • Peschanaya River and pit water;
- • the Severnaya Dvina, including its tributaries Pinega River and Sotkas River as well as local bog water.
Samples from the Yukova (zone 1) were collected from small streams or rivers and stagnant water (e.g., ice, pit water; Vasyukova et al., 2010). The Peschanaya (zone 2) is a pristine river draining to the Kuloy estuary of the White Sea. Water samples were collected during August 2006 and were affected by the input of peat bogs and swamps. The Severnaya Dvina (zone 3) was sampled in 2007 during three contrasted hydrological regimes: at the end of February, representing the base-flow winter conditions; in the beginning of May, when most of the thawing occurred; and the middle of June, at the beginning of summer base-flow conditions (Pokrovsky et al., 2010). In general, the spring flood (snow melt) lasts from 30 to 50 days and contributes about 60% of the annual water flux.
Additional river, stream and meltwater samples from Alaska were analysed as part of the sample set previously reported by Schroth et al. (2011) (Table S2, Supplementary material). Four broad classes of tributaries representative of main landscape of the Copper River watershed were sampled in August and October 2008, and include:
glacial tributaries that are milky brown in appearance, indicative of extremely high suspended sediment loads corresponding to the contribution of glacial meltwater;
proglacial tributaries fed by lake developed at the terminus of the glacier;
boreal forested montane streams that are not glaciered and have relatively low suspended sediment loads;
boreal forested “blackwater” tributaries draining large lowland areas in the Copper River basin and delta, with high concentrations of organic compounds. All samples from glaciered catchments were collected under peak glacial melt in August 2008, while forested catchments were sampled concurrently, but under summer base-flow conditions due to the lack of input from glacial ice in those systems.
3 Methods
The full description of the sampling and filtration methods and the geochemical data for the Ob’, Lena, White Sea area and Alaska Rivers are presented in the supplementary online materials. Fe isotopes and elemental analyses were performed on a range of suspended, dissolved and colloidal fractions filtered through 2.5 μm to < 1 kDa.
4 Results
4.1 Comparison of ultrafiltration systems
It is well recognized that ultrafiltration techniques may induce analytical artifacts due to charge separation, diffusion and clogging of the filter membrane (Dupré et al., 1999; Viers et al., 1997). Potential Fe isotope fractionation artefacts have been already discussed in previous studies (Ilina et al., 2013). As shown in Fig. 2, colloid sizes < 10 kD and < 1 kD separated using pressure ultrafiltration (UF) show an enrichment with heavy Fe isotopes by 0.26 to 0.37‰ compared with colloid sized separated through dialysis membrane (Dial) (Spectra Por 7) via passive diffusion. Hence, it is possible that separation by dialysis enriches the filtrate solution in light Fe isotopes, which is expected during diffusion mechanisms. However, this difference is of secondary importance when compared to the overall range of δ56Fe observed between dissolved Fe (< 0.2 μm) and colloidal Fe in most samples (e.g., #23, Y-3, Y-1, Y-5). In addition, because of the differences of techniques and potential filter clogging effects, dialysis and pressure ultrafiltration may not separate the same types of colloids.

δ56Fe and Fe/Al (g/g) ratios as a function of pore size in filtrates of the White Sea river system for zone 1 (Ruiga #9 and Ladreka #23 and Yukovo area Y-1 to Y-5) and zone 2 (Severnaya Dvina A-3, A-18, A-19 and Pinega A-7). The location and additional data on the samples are given in Tables S1 and S4.
4.2 Fe isotope composition of waters from White Sea area
In the Yukovo system, winter δ56FeDFe values show systematically positive values from 0.24‰ for sample Y-3 to 0.79‰ for sample Y-1 (Fig. 2). Colloidal (< 100 kDa and < 10 kDa) and truly dissolved or soluble (i.e. low molecular weight) fractions (< 1 kDa) of samples Y-1 and Y-3 also show systematic enrichment with heavy Fe isotopes relative to DFe (increase by up to 0.79‰ for Y-1 and 0.62‰ for Y-3). The enrichment with heavy isotopes is also associated with a drastic decrease of Fe concentration between DFe and soluble fraction (from 535 to 25 μg/L for Y-1 and 1117 to 46 μg/L for Y-3). Stagnant water (sample Y-4) and meltwater (sample Y-5) show lighter δ56Fe values for colloidal fractions, with δ56FeFeD values as low as –1.29‰ and –0.83‰, respectively (Fig. 2). It is important to note that Y-4, which corresponds to stagnant water trapped between two ice layers, also yields the highest Fe and Mn concentration and the most negative δ56Fe values for both suspended and dissolved pools (Table S4). Other samples from the same area (Ruiga #23 and Ladreka #9) recovered in the summer show a similar trend toward heavier δ56Fe values for smaller colloidal pools, although lighter δ56Fe values (down to –0.07‰) are observed for the < 1 kDa fraction in the Ruiga (Fig. 2). The Peschanaya waters (zone 2) yield δ56FeDFe values between –0.3 and –0.24‰ (samples s-32 and s-40, respectively), with slightly heavier value measured for truly dissolved Fe (δ56Fe = –0.07‰, Table S4).
The Severnaya Dvina (zone 3) was sampled over contrasting hydrological conditions. In general, this river is characterized by lower DOC contents, showing a significant increase during the spring period, consistent with the release of organic-rich materials during high flow. In contrast, Fe concentrations appear unrelated to changes of hydraulic regimes. δ56FeDFe yields near-zero values during base flow (i.e. –0.01‰ for samples A-3 and A-28), while heavier δ56FeDFe values up to 0.55‰ (sample A-18) are obtained during high flows. Similar increases in δ56FeDFe values during high-flow periods are also observed for the Pinega River, while identical values were obtained between high and base flow in the Sotka River. Colloidal fractions in rivers (A-3, A-18, A7) generally show a trend toward heavy Fe isotope enrichment with smaller-size colloids (Fig. 2).
4.3 Alaska rivers
Preliminary δ56FeDFe values of rivers from the Copper River watershed have been previously presented in Schroth et al. (2011). Additional values reported here include δ56FeDFe measured on a larger set of rivers and for different filtration pore size (i.e. 0.45 and 0.02 μm filters) (Fig. 3). In glacial and proglacial lake-fed tributary systems, the Fe isotopic signatures are similar to crustal values defined as 0.09‰ (±0.1‰) (Beard et al., 2003; Dauphas and Rouxel, 2006). In contrast, the boreal-forested systems display much lighter δ56Fe values down to –1.73‰, which also correspond to higher concentrations of DOC (Schroth et al., 2011).

δ56Fe and Fe concentrations for dissolved (< 0.45 and 0.22 μm) and soluble (< 0.02 μm) fractions from different tributaries of the Copper River watershed, including glacial, proglacial lake fed, boreal blackwater, and boreal montane. The location and additional data on the samples are given in Tables S1 and S5.
4.4 Fe isotope composition of large Arctic rivers
Time series samples of the Ob’ and Lena rivers were collected to capture the transition between base flow and high flow (Fig. 4; Table S3, Supplementary material). In the Arctic environment, the water discharge peak lasted a few hours to several days, leading to an extremely small sampling window. For the Lena River, sampling started just before the peak of discharge while for the Ob’ River, discharge rates remained essentially constant, suggesting that the system returns rapidly to low-flow conditions. Average δ56FeDFe values of both rivers are essentially identical within uncertainty, yielding δ56FeDFe = –0.11 ± 0.13‰ (2sd, n = 15 for the Lena, and n = 20 for the Ob’). The total range of δ56FeDFe is restricted to 0.23‰ for the Lena River and 0.30‰ for the Ob’ River, showing a lack of relationships between Fe isotope composition and discharge evolution. For the Ob’ River, lighter δ56FeDFe values (from –0.29 to –0.10‰) are measured at the beginning of the sampling period when the discharge rate is slightly higher (from 3.2 to 3.0 km3/d), but this trend is not observed for the Lena River where changes of discharge rates are larger (from 2.1 to 9.5 km3/d).

Daily discharge measured at Salekhard and Kyusyur stations for Ob’ and Lena respectively, δ56Fe values of the Ob’ and Lena filtered water collected as part of the Student Partners Project.
5 Discussion
Variable δ56FeDFe values have been already reported in rivers from temperate and tropical environments, with a total range from –0.7 to 0.8‰ (see Figure S1, supplementary material). Although early studies proposed that dissolved Fe in rivers had δ56FeDFe values lighter than those of the bulk continental crust (Bergquist and Boyle, 2006; Fantle and DePaolo, 2004), more recent studies have identified ubiquitous heavy δ56FeDFe values in organic-rich rivers of temperate region and in the Arctic (Escoube et al., 2009; Ilina et al., 2013). The analysis of our new dataset supports the recent study of Ilina et al. (2013), confirming that DFe and colloidal Fe in Arctic rivers display an extreme range of δ56FeDFe from –1.4‰ to 2‰. This suggests a dynamic and complex partitioning of Fe isotopes between particulate, colloidal and “dissolved” fractions, which may be unique to high-latitude river networks.
In the Copper River watershed in Alaska, systematically lighter δ56FeDFe values are commonly observed in organic carbon- and Fe-rich boreal rivers, while glacial rivers do not show significant fractionation relative to bulk crust (Figs. 3 and 5). An important exception includes a small river draining montane boreal forest (i.e. Tractor creek) showing heavier values up to 0.68‰ (Fig. 3). Glacial rivers characterized by near crustal δ56Fe should mainly reflect the contribution of particles and colloids derived from physical erosion, where the mechanical transport of lithogenic materials should proceed with minimal Fe isotope fractionation. In this case, the slight enrichment with heavy isotopes observed in some glacial rivers could be attributed to the alteration of specific lithologies, such as shales recognized as potential source of heavy Fe (Yesavage et al., 2012) or isotopically heavy crystalline rocks such as granite (Poitrasson and Freydier, 2005).
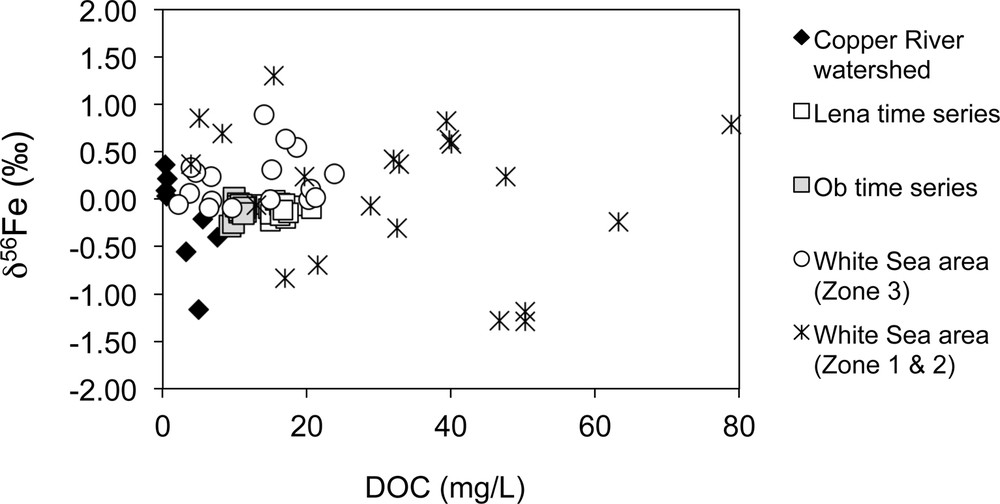
δ56Fe values and DOC concentrations of soluble and colloidal fractions from studied Arctic and sub-Arctic watersheds.
The lighter δ56FeDFe values of boreal forested rivers has been previously interpreted to reflect either the contribution of groundwater and/or soil water-derived Fe with DOC derived from organic matter decomposition (Schroth et al., 2011). It has been experimentally demonstrated that equilibrium Fe-organic complexation would favor heavy Fe isotopes in organically-bound Fe (Dideriksen et al., 2008), while kinetic mineral dissolution in the presence of Fe-chelating organic ligands would favor the release of light Fe into solution (Brantley et al., 2001; Kiczka et al., 2010a). Plant uptake may also favor light Fe isotopes that may be released to rivers after the decomposition of soil organic matter (Kiczka et al., 2010b). Additional constraints on the origin of isotopically light Fe in organic-rich rivers may be derived from our new data from ice meltwater and time-series, as discussed below.
Several lines of evidence suggest that dissolved Fe in ice meltwater is enriched with light isotope. In the Alaska system, glacial meltwater (sample #St32) shows δ56FeDFe = –0.81‰, while labile (< 10 kDa) and fine particulate Fe (< 2.5 μm) from water trapped in ice from the White Sea area (sample Y-4) show δ56Fe ranging from –1.04 to –1.28‰. Light δ56FeDFe values have been also reported in Antarctic sea ice particulate matter and dissolved Fe, with values probably lower than –1.5‰ (de Jong et al., 2007). These light values have been interpreted as reflecting the presence of heterotrophs in the upper layers with predominantly flagellates and bacteria. Here, we propose instead that the generally light δ56Fe in meltwater is mainly the result of redox effects rather than of biological uptake. The overall enrichments with Fe and Mn in sample Y-4, associated with the lightest δ56Fe values (Table S4), suggest a contribution of anoxic to suboxic water. Sample Y-4 is trapped between two ice layers and is therefore isolated from the atmosphere, allowing reducing conditions to build up, promoting the release of labile Mn(II) and Fe(II) during the degradation of particulate organic matter by heterotrophic organisms. Considering the ca. 3‰ fractionation factors between FeII and FeIII (Welch et al., 2003; Wu et al., 2011), Fe(II) is expected to be enriched with light isotopes relative to Fe(III) remaining in the suspended particles. Similar processes involving Fe redox cycling have been well identified during diagenetic reactions in marine sediments and porewater (Homoky et al., 2009; Rouxel et al., 2008; Severmann et al., 2006) as well as during redox-controlled release of Fe in soils (Schuth et al., 2015), which also likely occurs during the formation of meltwater (Bhatia et al., 2013).
It has been recently shown that glacial runoff may provide a significant source of bioavailable iron to surrounding coastal oceans as a result of ice melting (Bhatia et al., 2013). Two mechanisms have been proposed to explain the increase of dissolved Fe concentration in runoff meltwater, including the contribution of Fe-rich hypoxic or anoxic water in the subglacial drainage system or an increase of DOC concentrations. Based on our results, it seems that Fe isotope systematics may provide means to distinguish between these two mechanisms. When Fe is bound to organic ligands (i.e. DOC), it should be enriched with heavy isotopes (e.g., Dideriksen et al., 2008). In contrast, when Fe is released from oxygen-depleted meltwater, it should be enriched with light isotopes.
The small organic-rich rivers from the White Sea watershed (Severnaya and Pinega Rivers) show heavier Fe isotope values during high discharge periods and for small-size colloids (Fig. 2, Table S4). Differences in δ56FeDFe of up to 0.6‰ and 0.7‰ have been reported for Severnaya and Pinega Rivers between low- and high- flow. Soluble fractions (< 1 kDa) also show systematic enrichment with heavy Fe isotopes relative to DFe (e.g., increase by up to 0.79‰ for sample Y-1). As discussed previously (Ilina et al., 2013), heavier δ56Fe values in small Arctic rivers should mainly reflect a larger contribution of organic-rich small colloids that undergo seasonal recycling and mixing between different colloidal sources. Heavier δ56Fe values are therefore consistent with the complexation of Fe(III) with strong organic chelates, as confirmed experimentally by Dideriksen et al. (2008), although sedimentary rock weathering (e.g., shale) may also provide an alternate source of isotopically heavy colloidal Fe in rivers due to incongruent dissolution mechanisms (Yesavage et al., 2012). The later hypothesis may also explain the significant fractionation of Fe vs. Al between different colloid sizes. The overall decrease of Fe/Al ratios for smaller colloid size with a concomitant increase in δ56Fe (Fig. 2) suggests the existence of Fe-depleted and isotopically heavy reservoir generated by multiple alteration stages. This hypothesis is also consistent with the lack of inverse correlation between δ56Fe and DOC (or DOC/Fe) (Fig. 5), suggesting rather a large range of δ56Fe values in organic-rich colloids from the White Sea watershed).
By comparison, the relatively constant δ56Fe values of the Lena and Ob’ Rivers over time (δ56Fe = –0.11 ± 0.13‰) contrast with the large variability observed in smaller river systems, either from temperate, tropical or boreal regions. Considering that Ob’ and Lena samples show similar or even higher enrichment with DOC (10 to 20 mg C/L) than organic-rich rivers from boreal-forested rivers (Schroth et al., 2011) (Fig. 5) and even tropical rivers (Bergquist and Boyle, 2006), the small Fe isotope fractionation of ca. −0.2‰ relative to bulk crust is surprising. Presumably, the release of Fe-rich colloids during the peak discharge does not allow significant particulate-dissolved isotope exchange, as observed in smaller organic-rich riverine systems. This suggests the absence of a significant contribution of a fractionated reservoir in larger Arctic rivers derived from anoxic swamps and meltwater or from plant litter decay in summer. Alternatively, this could be also interpreted as an integrated signal from these fractionated reservoirs whose relative contributions stay similar throughout the hydrologic year. This contrasts with smaller rivers where shorter flow paths would produce variable mixing ratios of these sources and therefore, more variable Fe isotopic compositions. Alaskan rivers also support this hypothesis since the Copper River shows homogeneous δ56Fe values despite the variable δ56Fe values measured in its tributaries.
The variations of dissolved and particulate δ56Fe values have been generally attributed to distinct weathering processes and environmental parameters (Pinheiro et al., 2013, 2014; Poitrasson et al., 2014; Song et al., 2011). It has been also proposed that Fe isotope composition of suspended particulate matter is possibly linked to climatic conditions, with high latitude rivers exhibiting mostly positive δ56Fe values, while tropical rivers showing strongly negative Fe isotopic signatures (Pinheiro et al., 2014). Our new data suggest that this model does not necessarily apply to large rivers such as Ob’ and Lena. Isotopically light organic-rich rivers may also occur in sub-Arctic climate as those reported in the Copper River watershed in Alaska.
6 Concluding perspectives on the global flux of Fe isotopes in Arctic environments
The impact of global warming on permafrost degradation in the Arctic has received considerable attention (Dittmar and Kattner, 2003; Frey and McClelland, 2009; O’Donnell et al., 2012; Romanovsky et al., 2010). Permafrost-driven changes in watershed hydrology have been invoked to account for the increased flux of dissolved organic carbon from terrestrial to aquatic and marine ecosystems, in relation to basin-wide permafrost thaw and an increase in groundwater contribution in base flow. An important feature of all boreal catchments is the large flux of dissolved and particulate matter and especially organic carbon occurring during a relatively short high-flow period of snowmelt (Holmes et al., 2012; Pokrovsky et al., 2010).
Considering the combined annual water discharge of the Ob’ and Lena of 1015 km3/yr (Holmes et al., 2012), corresponding to ca. 40% of the global riverine flux in the Arctic Ocean, our study allows the first estimation of the Fe isotope composition of riverine dissolved Fe flux in the Arctic Ocean. To our knowledge, the annual fluxes of Fe from the Ob’ and Lena have not been reported in previous studies. Hence, at a first approximation, we consider that Fe flux is proportional to DOC flux, with relationships of Fe/DOC = 21 ± 6 (g/kg) for Lena river and 61 ± 8 (g/kg) for Ob’ river (Table S3). Although Fe enrichment with rivers is often associated with organic or humic-rich colloids (Allard et al., 2004), the long-term relationship between DOC and Fe in these rivers should be however used with caution. Using the annual DOC fluxes determined in previous studies (Holmes et al., 2012), we determine a total annual flux of dissolved Fe of 84 ± 25 (109 g/yr) for Lena river and 252 ± 34 (109 g/yr). By normalizing δ56FeDFe to Fe fluxes, we further determine the annual discharge of Fe having δ56FeDFe = –0.110‰ for Lena river and δ56FeDFe = –0.112‰ for the Ob’ River. These values suggest that large rivers, contributing to the largest input of freshwater into the Arctic Ocean, have very homogeneous δ56FeDFe values, slightly enriched with light isotopes by 0.2‰ relative to bulk continental crust. The similarities of average δ56FeDFe between these two large rivers is interesting considering their contrasted permafrost coverage, totalling 4% for Ob’ river and 90% for Lena river (Holmes et al., 2012). Although the spring flow period is not the most affected by permafrost thaw, our results argue against the significant influence of permafrost degradation on Fe isotope composition of DFe in sub-Arctic rivers.
In contrast, smaller Arctic river systems show much larger spread in δ56FeDFe values, with a marked enrichment with heavy Fe isotopes up to 0.55‰ during spring flood period and for small colloids. The Severnaya Dvina is the largest European sub-Arctic river, contributing to 4% of the total water discharge to the Arctic Ocean (e.g., Pokrovsky et al., 2010). The estimated total annual DFe flux from the Severnaya Dvina has been previously determined to 53 ± 16 109 g/yr (Pokrovsky et al., 2010), which is more than 50% of the total Fe flux of the Lena. Hence, smaller Arctic rivers may contribute disproportionately to the input of DFe in the Arctic Ocean, while showing strongly fractionated (i.e. heavier) Fe isotope values. Since the Severnaya Dvina drains both silicate-bearing and carbonate rocks, a similar source of heavy DFe may be commonly observed in other remote rivers of the Arctic. It has been also recently demonstrated that the most labile Fe fraction (i.e. < 1 kDa) in Arctic rivers increases its concentration by a factor of 5 during estuarine mixing (Pokrovsky et al., 2014). Hence, the labile and potentially bioavailable Fe in small organic-rich Arctic and sub-Arctic rivers may provide an important source of both isotopically light and heavy Fe to the Arctic Ocean, with δ56Fe values as high as 2.7 ‰ (Ilina et al., 2013) and as low as –1.7‰.
We interpret the striking contrast of Fe isotope signatures between large versus smaller organic-rich arctic rivers to be influenced by the fact that smaller rivers tend to have more northerly watersheds, therefore integrating a smaller number of Fe sources. Hence, element source and biogeochemical cycling may be considerably different than for the larger rivers, such as Ob’ and Lena, whose watersheds extend much farther south. Seasonal measurements of Fe and other element concentrations and speciation reveal the presence of two main sources of Fe that are preferentially mobilized and disproportionately influence riverine Fe loads under different conditions during the high-latitude hydrologic year (Pokrovsky et al., 2010): (1) deep groundwaters poor in organic matter and (2) colloids and organic-rich surficial soil waters. These sources should have contrasted δ56FeDFe values, with isotopically light δ56FeDFe values for groundwater-derived Fe on the one hand, and isotopically heavy δ56FeDFe values for soil-derived organic-rich colloids on the other hand. The impact of climate change in the Arctic may therefore differ among these classes of rivers and Fe sources, producing contrasted and evolving Fe isotope composition for global Fe delivery in the Arctic Ocean.
Acknowledgments
This work was supported by the National Science Foundation (OCE 0550066), WHOI Arctic Research Initiative, Labex Mer (ANR-10-LABX-19-01), and FP7 (#247837), RFFI No 13-05-00890, 14-05-98815 and BIO-GEO-CLIM No 14.B25.31.0001 grants. This work would not have been possible without the initial collaborations with Bernhard Peucker-Ehrenbrink and John Crucius. Christian Miller. We also thank Lary Ball, Jurek Blustajn, Fanny Chever, Emmanuel Ponzevera, and Maureen Auro for their technical support. We thank Sébastien Bertrand, Bernhard Peucker-Ehrenbrink, Valier Galy, Catherine Jeandel and Francois Lacan for helpful discussions and Marc Benedetti and Vincent Busigny for helpful comments on the manuscript.