1. Introduction
Whatever their nature, chemical composition or physical state, fluids play key roles in the evolution of the Earth’s lithosphere, particularly regarding sediment diagenesis, volcanic activity, hydrothermal alteration, metamorphism, ore deposit formation, among many other phenomena. Fluids are also important with regard to deformation, especially brittle deformation. In the upper brittle crust, fluids can circulate in deforming zones, namely fracture zones or fault zones, and can chemically or mechanically interact with host rocks. Such interactions are also conceivable in subduction zones, where the upper part of the subducting plate keeps a brittle behavior down to hundreds of kms of depth.
The role that fluids exert on rock deformation is of paramount importance for human societies. Indeed, fluid extraction (e.g., hydrocarbon industry) or fluid injection (geothermal energy, waste water disposal, gas storage) trigger or enhance seismic activities in otherwise aseismic or moderately active regions.
This review aims at bridging the gap between the mechanical interactions between fluids and rocks on one hand and the structural analysis of deformed rocks from the scale of the hand sample to that of the deforming plate boundaries. For space reasons, the chemical effects of fluids, although of considerable importance, will not be addressed here.
2. The mechanical interaction between fluids and rocks
2.1. The development of abnormally high pore fluid pressures in the upper crust
Production of fluid in depth, for instance by magmatic activity or by metamorphic or metasomatic reactions, can result in an influx of fluids. If there is a permeability barrier that prevents or hinders fluid escape, then the pore fluid pressure pf will increase. This increase can also be generated by pore volume reduction in low-permeability rocks. For instance, elastic crack relaxation following an earthquake (seismic pumping, see below Section 2.6) or inelastic pore compaction controlled by the applied shear stress (shear-enhanced compaction, see below Section 2.7) are two important mechanisms responsible for pore volume decrease. Beside such earthquake-related mechanisms, pore compaction can also be achieved during diagenesis [Walder and Nur 1984; Wang et al. 2022]. As attested by measurements in deep boreholes, supra-hydrostatic pf values actually develop below impermeable barriers or seals, and can reach the value of the lithostatic stress or even exceed it [Yerkes et al. 1985; Powley 1990; Neuzil 1995; Zencher et al. 2006].
2.2. Intact rock fracturing, stress corrosion, hydrofracturing
Classical rupture criteria for intact rocks in the upper crust are inspired by experimental rock mechanics and state that, as long as the stress tensor (whose components are 𝜎1, 𝜎2, and 𝜎3, with the convention 𝜎1 > 𝜎2 > 𝜎3) acting on the rock does not satisfy any criterion, no fracture will form. To the contrary, once the stress tensor satisfies the criterion, microscopic fractures will propagate through the rock, eventually leading to the formation of a macroscopic fracture or fault.
Two rupture criteria are commonly used in the analysis of intact rock failure. The simplest is the linear Mohr–Coulomb criterion, which postules that the propagation of macroscopic fractures happens when
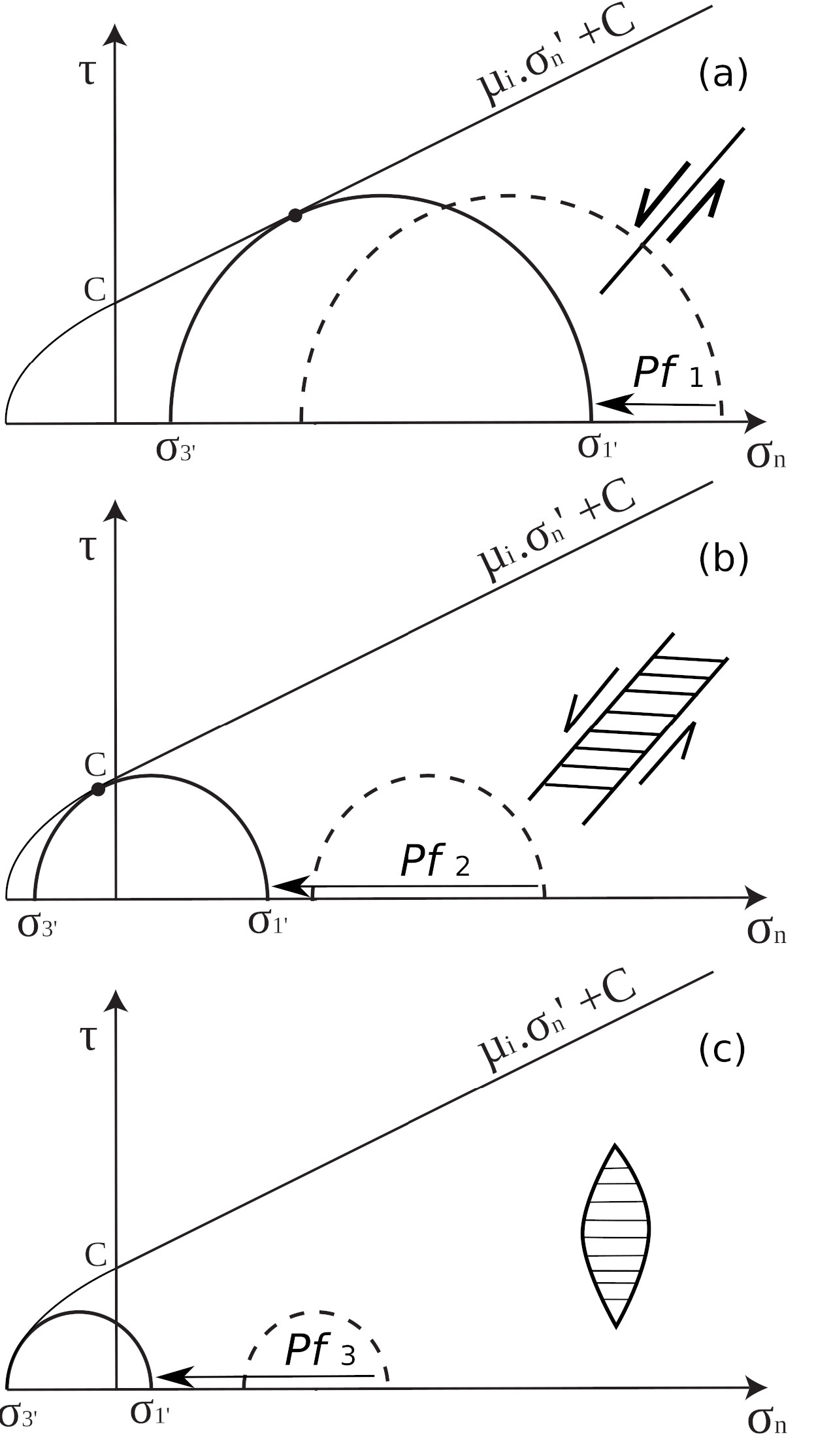
The role of pore fluid pressure in fracturing intact rocks as representated in the Mohr space. The intact rock rupture criterion is composite, that is, it consists of the Griffith criterion for the negative normal stresses, and of the linear Mohr–Coulomb criterion for the positive normal stresses.
The role of pore fluid pressure in fracturing intact rocks as representated in the Mohr space. The intact rock rupture criterion is composite, that is, it consists of the Griffith criterion for the negative normal stresses, and of the linear ... Lire la suite
In the absence of pore fluid in the rock, the convergence between the tensor and the criterion can be achieved only by increasing the differential (deviatoric) stress 𝜎D = 𝜎1 − 𝜎3, that is, either by decreasing 𝜎3, by increasing 𝜎1, or by acting on both 𝜎3 and 𝜎1. If a fluid is present inside the rock (so-called pore fluid), then two processes can lead to the formation of macroscopic fractures. In the first process, called stress corrosion, the fluid will chemically react at the atomic scale with the rock at the tip of a pre-existing loaded crack, so that the critical stress intensity factor at this location gets lower and lower. When the stress intensity factor at the tip of the crack reaches the fracture toughness of the altered rock, the fracture will propagate. This phenomenon is called sub-critical crack growth [Anderson and Grew 1977; Atkinson 1984; Brantut et al. 2013]. The second process requires that the pore fluid is elevated at a pressure pf. If so, the components 𝜎i of the tensor will then be changed into effective components
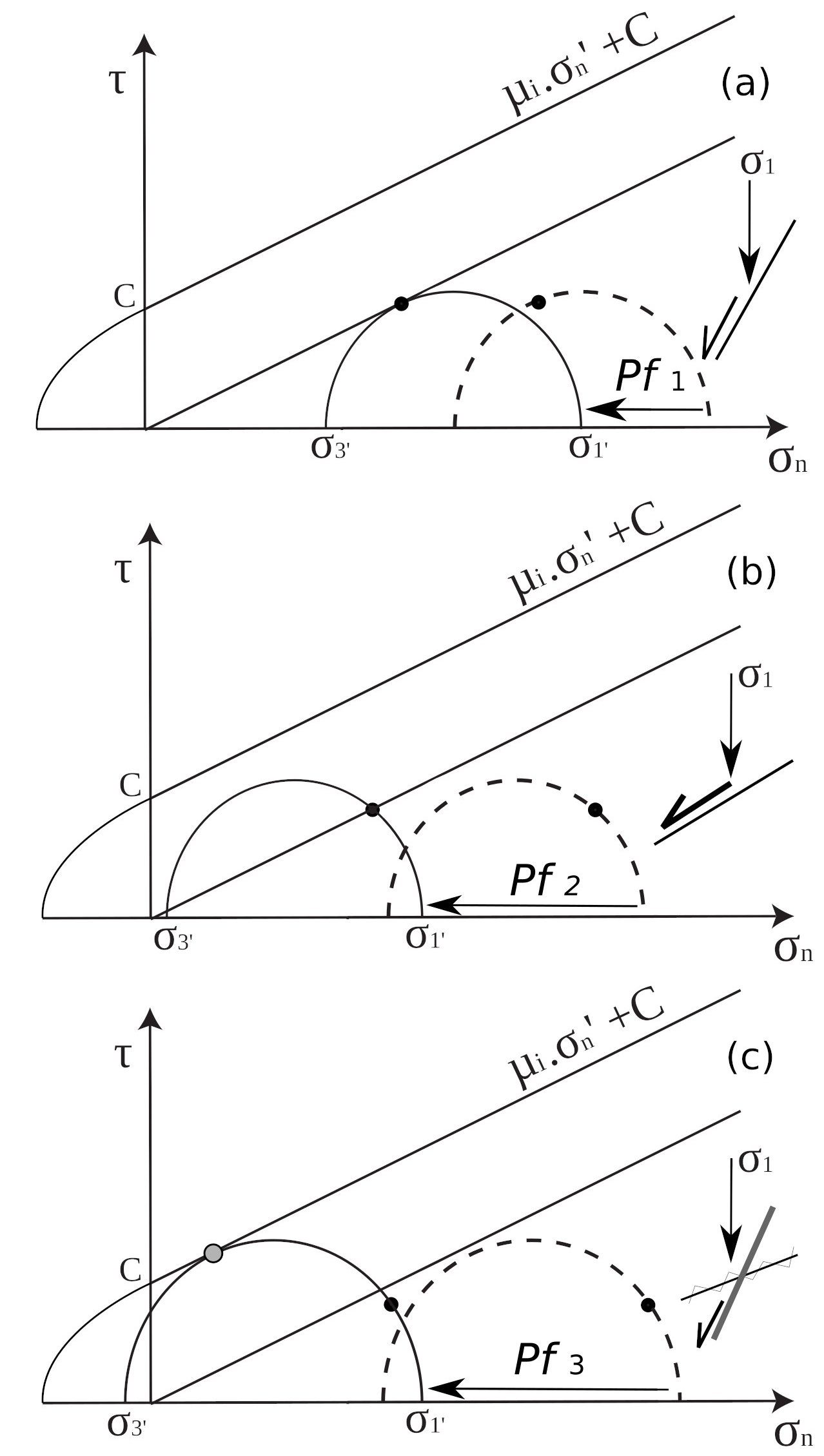
Competition between reactivation of pre-existing fractures and formation of a new fracture [after Handin 1969; Sibson 1985]. The failure envelope for intact rock follows a composite Griffith–Mohr–Coulomb criterion. The reactivation envelope is linear, and its cohesion is neglected.
Competition between reactivation of pre-existing fractures and formation of a new fracture [after Handin 1969; Sibson 1985]. The failure envelope for intact rock follows a composite Griffith–Mohr–Coulomb criterion. The reactivation envelope is linear, and its cohesion is neglected. ... Lire la suite
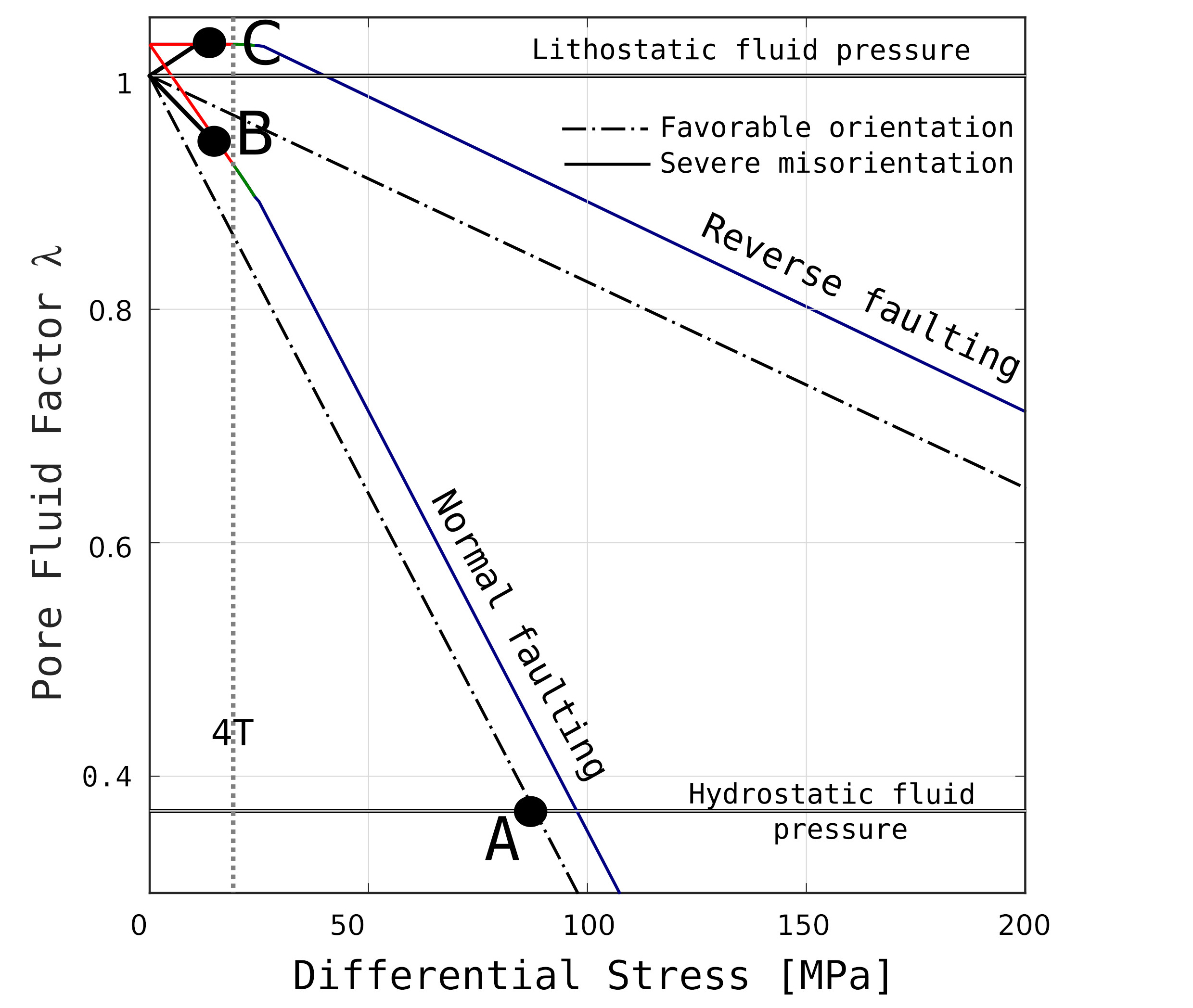
Failure mode diagram for reverse and normal faulting based on the Mohr–Coulomb theory. Red, green and blue lines correspond respectively to pure extensional (dilatant) veins, hybrid veins and shear fractures. Oblique black lines correspond to the reactivation conditions for a favorably oriented fracture or a severely misoriented fracture. The diagram is drawn for a depth z of 7 km, a rock density 𝜌 of 2.7, a friction coefficient 𝜇 of 0.75 and a tensile strength T of 5 MPa. See text for explanation of points A, B and C. Masquer
Failure mode diagram for reverse and normal faulting based on the Mohr–Coulomb theory. Red, green and blue lines correspond respectively to pure extensional (dilatant) veins, hybrid veins and shear fractures. Oblique black lines correspond to the reactivation conditions for a ... Lire la suite
Dilatant breccias form when the three effective principal stresses are negative (
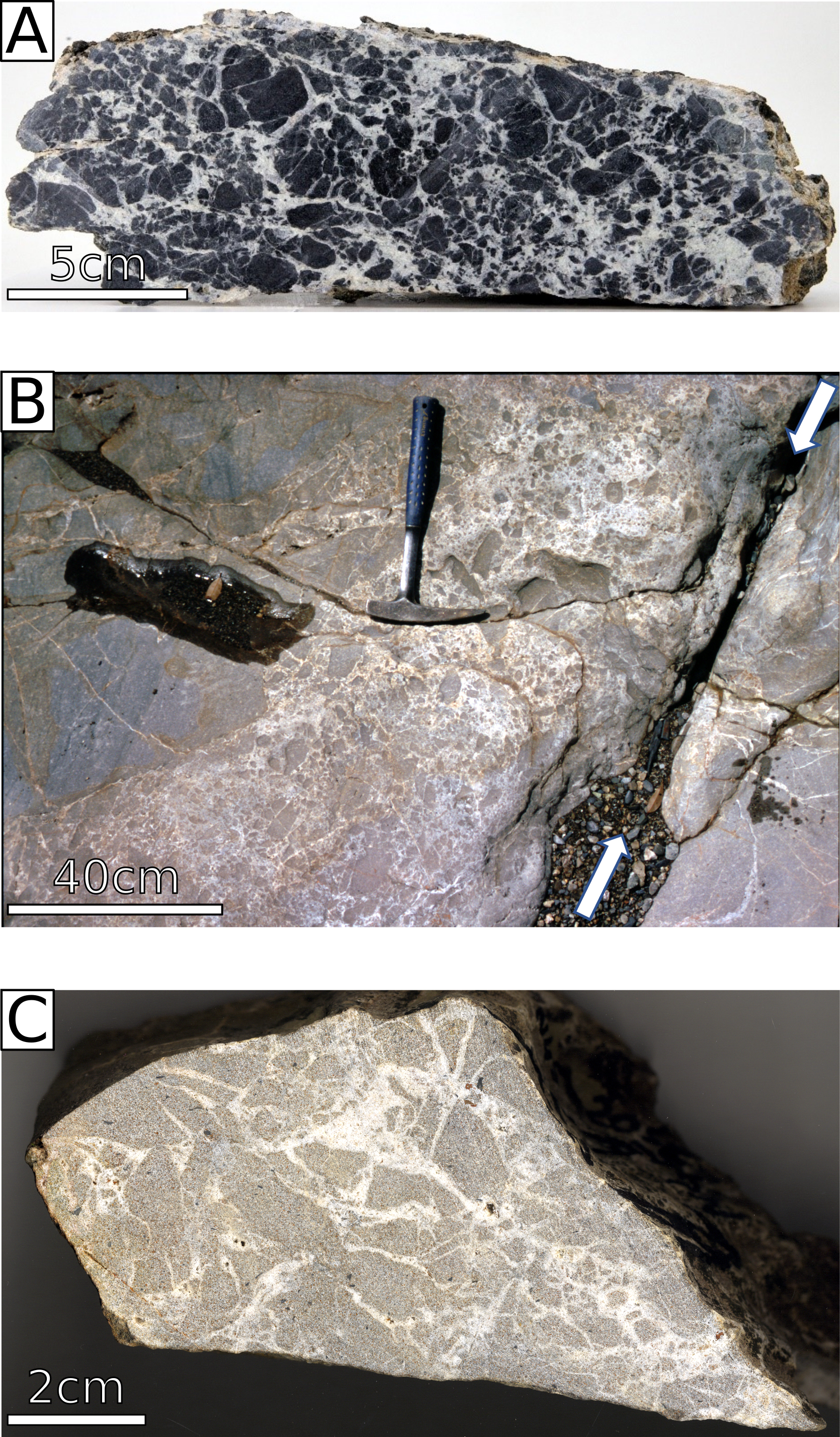
Purely dilatant breccias (hydraulic breccias) suggesting a hydrostatic stress state (
Purely dilatant breccias (hydraulic breccias) suggesting a hydrostatic stress state (
Mineralized extensional joints (tension gashes) are typical and widespread geological structures resulting from failure of intact rocks by pure extension (Figure 1c). Pure extension is achieved by the condition
Shear fractures result from a combination of extension and shear (hybrid failure). They classically form conjugate systems of en échelon fractures (Figure 5), where the long axis of the mineral fibers indicates the combination of extension and shear (Figure 6).
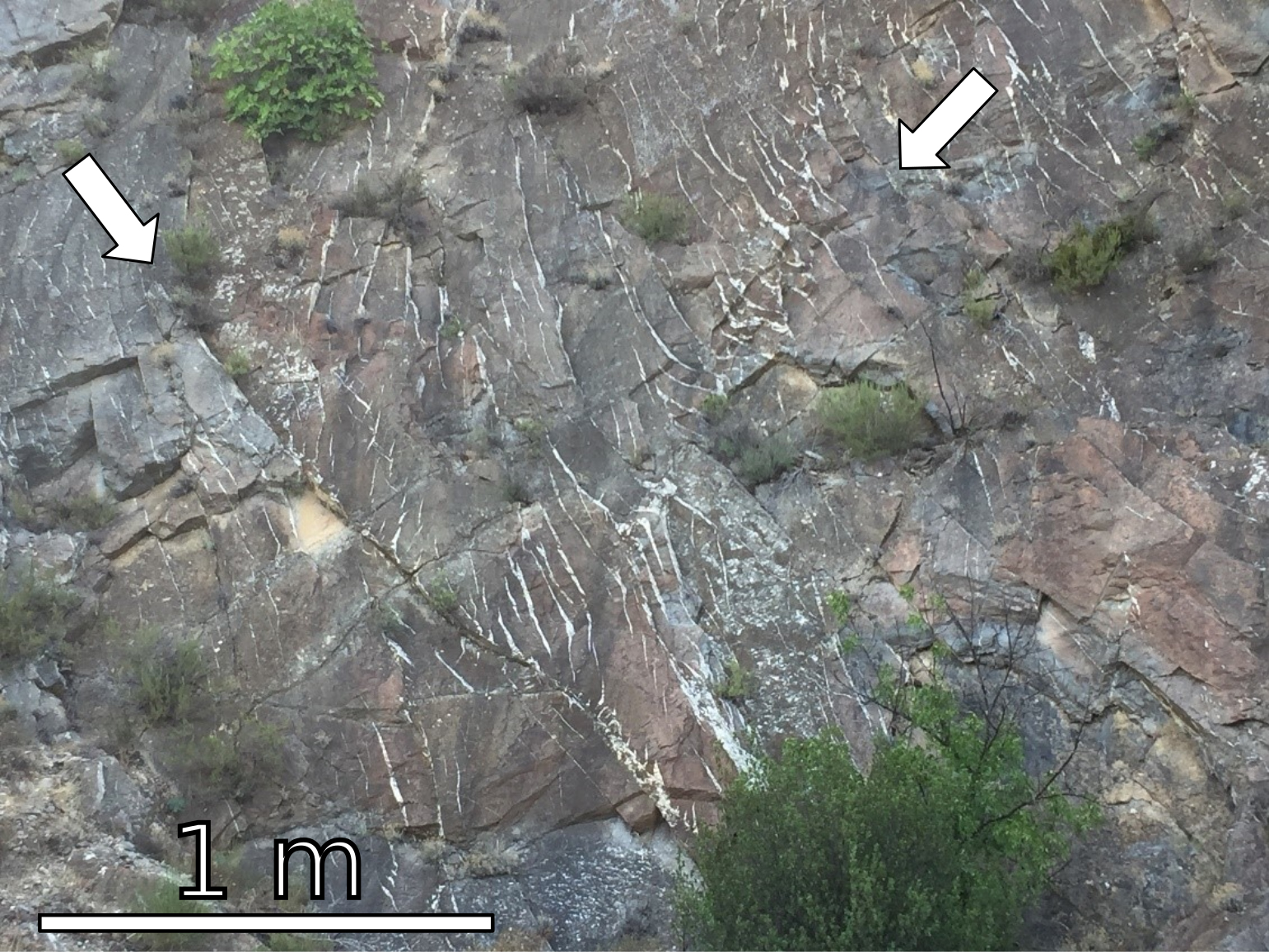
Calcite-filled mode I and hybrid mineralized fractures associated with conjugate faults (arrows). The fractures are perpendicular to the steeply dipping bedding surface. Cretaceous limestones, Pyrenean foreland, Corbières, France (height of photograph: 2 m).

Mode I quartz-filled fracture in low-porosity Oligocene sandstones, Shimanto accretionary prism, Japan. The quartz fibers show an S shape, suggesting a component of displacement parallel to the fracture. Thickness of the vein: 15 mm.
2.3. Fracture reactivation vs. fracture neoformation
Unlike in laboratory experiments, rock masses in the brittle crust are fractured. Therefore, it is not the intact rock strength that will dictate the stability of rock masses, but rather the strength of discontinuity (fault or fracture) surfaces [Byerlee 1978]. Intact rock rupture criteria are replaced by more realistic reactivation criteria. The simplest and most widely used criterion is the Mohr–Coulomb reactivation criterion [Handin 1969], quite similar to the Mohr–Coulomb criterion for the intact rock, and which can be written as
2.4. The reactivation of moderately to severely misoriented fault surfaces
In the brittle crust, the experimentally demonstrated preference of fracture neoformation over preexisting misoriented fracture reactivation does not seems to be a general rule. Indeed, in many instances, moderately to severely misoriented fault surfaces are reactivated, while no propagation of new surfaces is observed. Several mechanisms are proposed to solve this paradox, among which the most frequently invoked are as follows.
(1) The fault zone consists of a continuous layer of weak rocks, for instance rocks whose rheology can be regarded as plastic, visco-elastic, or elasto-plastic. This is the case with clay-rich or evaporite-rich strata (so-called décollement layers). Such a weak and continuous décollement layer will allow the displacement of allochton units over significant distances whatever the orientation of the fault zone with respect to the stress axes is favorable or not [Davis and Engelder 1985; Weijermars et al. 1993; Costa and Vendeville 2002; Vendeville et al. 2017].
(2) The fault surface is coated by low-friction (𝜇 < 0.2) material such as low-friction clay (e.g., montmorillonite) or talc [Morrow et al. 1992, 2017; Moore and Lockner 2011; Boutareaud et al. 2012; Chen et al. 2017]. Leaving aside the stress corrosion process mentioned above, which concerns the nucleation of a mesoscopic fracture rather than reactivation of a macroscopic fracture or fault, fluids can weaken a fault by chemically reacting with the rocks or minerals along the damage zones or core zones of the fault. The chemically produced minerals can be frictionally weaker (so-called reaction softening). Examples of chemical weakening of fault rocks are described by White and Knipe [1978], Evans and Chester [1995], Wintsch et al. [1995], Gueydan et al. [2003], Collettini and Holdsworth [2004], Matsuda et al. [2004], Jefferies et al. [2006], Moore and Rymer [2007], Collettini et al. [2009b]. As indicated above, this reaction-softening effect will not be developed here.
(3) Along long-lived mature faults, damaged rocks inside the fault zone get their mechanical constants, such as their Young moduli or Poisson ratios, modified [Faulkner et al. 2006]. These modifications lead to a rotation of the stress axes in the vicinity of the fault [Healy 2008]. The angle between 𝜎1 and the fault surface can thus be decreased and a very unfavorably oriented fault can become favorably oriented for reactivation. The studies of Provost and Houston [2001], Hardebeck and Michael [2004], Famin et al. [2014] suggest that vertical or steeply dipping strike-slip faults such as the San Andreas fault in California or the Median Tectonic Line in Japan can be reactivated following a rotation of the horizontal 𝜎1 axis to a more favorable value.
(4) The last mechanism, which is the main purpose of this paper, calls for the development of abnormally high pf, which will decrease the normal stress 𝜎n to the effective
2.5. The fault valve model
The fault-valve model, which is a consequence of the stress analysis of Sibson [1985], is based on the existence, in faulted regions, of fault planes moderately or severely misoriented with respect to the stress axes at the time of the fault activity [Sibson et al. 1988; Sibson 1989, 1990]. The model is based on the following assumptions: (1) the coefficient of friction on the preexisting (misoriented) fault is of “Byerlee” type, that is, its value is between 0.6 and 0.85; (2) the cohesion C of the surface is neglected; (3) the fluid does not chemically react with the rock, that is, only the fluid pressure pf plays a role.
The typical fault-valve behavior of a fault or fault system involves cyclical variations of pf. A cycle consists of four stages: (1) pf increases up to supra-hydrostatic values below a hydraulic barrier, (2) rupture of the fault plane following a Mohr–Coulomb type criterion [Sibson 1985], (3) fluid escape (upward or sideward) following increase of the rock permeability by fracturing and cataclasis associated with fault and hydraulic barrier ruptures, (4) fracture or pore sealing by mineral precipitation due to the sudden decrease of pf down to hydrostatic values. This last stage eventually results in a restauration of the former seal, thus bringing back the faulted rock mass to a state before stage (1), allowing a repetition of the cycle. Following the initial discovery of this mechanism in mesothermal gold-quartz deposits by Sibson et al. [1988], this behavior was recognized in ancient fault systems [Cox 1995; Robert et al. 1995; Hacker 1997; Nguyen et al. 1998; Sibson and Scott 1998; Faleiros et al. 2007]. The extensive damage to the rocks adjacent to the faults suggests that reactivation might have been seismogenic. The fault-valve model is proposed to account for present-day seismic reactivation of unfavourably oriented faults (see Section 4).
2.6. The seismic pumping mechanism
Seismic pumping provides a mechanism of formation of zoned mineralized veins along faults in the brittle crust by calling for fluid flow during earthquakes [Sibson et al. 1975; Kerrich et al. 1987]. The mineral zonation is interpreted as the result of repeated arrivals of fluids, each arrival leaving an imprint in the vein. In the pre-seismic stage, due to tectonic loading along the fault, dilatant structures such as tension cracks will create voids along and near the fault, aspirating nearby fluids. In the post-seismic stage, fluids will be expelled upwards or sidewards, before the cycle starts again. In itself, the seismic pumping mechanism does not increase directly the pf value, but contributes to it by injecting volumes of fluids from remote areas towards active fault zones. It can also be active during diagenesis in faulted sedimentary basins [Wood and Boles 1991].
2.7. Shear stress-enhanced compaction
In most cases, the central part, or core, of a fault zone is constituted by a weak material (clayey gouge, poorly consolidated breccia and so on). When tectonically loaded, a core material may undergo more compaction than the stronger surrounding rocks (damage zone, country rocks). If the core zone is (i) saturated by fluids and (ii) sealed (i.e., the fluid cannot escape), then tectonic load-induced compaction will increase pf [Byerlee 1990; Blanpied et al. 1992; Sleep and Blanpied 1992]. The pf increase will then decrease the effective normal stress
2.8. Thermal pressurization
Thermal pressurization, also called “dynamic” pressurization, concerns thin (a few millimeters to a few centimeters) fault core zones which are the site of coseismic slip along faults in the upper crust [Sibson 1973; Lachenbruch 1980; Mase and Smith 1987; Wibberley and Shimamoto 2005]. The phenomenon is based on a contrast between the thermal expansion of aqueous fluids trapped in the very-low-permeability fault core rock (clayey gouge in most cases) and the thermal expansion of the fault core rock matrix itself. During co-seismic, slip, the fluid-saturated rock will thermally expand due to shear heating, but fluids will expand in larger proportions than the pores. As long as the rock permeability remains low (e.g., in the absence of fracturing), the fluid cannot escape from the core zone and, following expansion, its pressure will increase drastically, resulting in a decrease of the normal stress acting on the surface.
The recognition of thermal pressurization processes during earthquakes or in the geological record is a challenge and, in addition to observations of natural microstructures [Ujiie et al. 2007, 2010; Boullier et al. 2009; Boullier 2011], must rely on experimental reproduction of seismic slip and on numerical modeling [Noda and Shimamoto 2003; Segall and Rice 2006; Ujiie et al. 2011; Boutareaud et al. 2008; Ferri et al. 2010; Kitajima et al. 2011; Viesca and Garagash 2015]. In addition to thermal pressurization strictly speaking, frictional heating can also lead to thermal decomposition of minerals such as phyllosilicates or carbonates (calcite, siderite), resulting in the release of fluids (H2O, CO2) that can then be pressurized [Brantut et al. 2008, 2010; Hirono et al. 2008; Ferri et al. 2010; Jeanne et al. 2014]. Frictional melting of the fault surface during co-seismic slip in carbonate-rich rocks can also result in a release of large amounts of CO2 following volatile exsolution from the melt [Famin et al. 2008]. In the thermal decomposition or exsolution processes, the released fluids are considered to be pressurized and can consequently decrease the normal stress acting on the faults. Some authors contend that so-called injected cataclasites or injected gouges may result from co-seismic thermal pressurization [Lin et al. 2013; Lin 2019].
3. Role of abnormally high pf in crustal tectonics
3.1. Role of abnormally high pf in thrust tectonics
In areas dominated by thin-skinned tectonics, thrust faults can accommodate significant (>10 km) horizontal displacements. To overcome the mechanical resistance of the allochtonous units to displacement, two mechanisms among the ones described above are frequently called for. The first mechanism (the translation made possible by weak ductile décollement layers) allows to account for the formation of several foreland thrust-and-fold belts such as the French-Swiss Jura belt or the Iranian Zagros belt [Jordan 1992; Sherkati et al. 2006; Sommaruga et al. 2017; Lacombe and Mouthereau 2002].
The other mechanism, that is, the translation of thrust sheets triggered or favored by supra-hydrostatic pf, is inferred or ascertained in various tectonic settings. Table 1 provides examples of studies that call for supra-hydrostatic pf to account for horizontal displacements along thrusts in collision belts or foreland fold-and-thrust belts. The supra-hydrostatic pf lowers
Examples of geological or geophysical evidence for displacement along low-angle reverse faults triggered or favored by supra-hydrostatic pore fluid pressures pf inside or near fault zones in collision belts or foreland fold-and-thrust belts, with pore fluid ratio 𝜆 estimates where available
Area | Name of structure | Evidence |
---|---|---|
Central Alps | Glarus thrust | Fracturing, veining, brecciation alternating with ductile deformation (𝜆 > 1)[1]–[4] |
Western Alps | Helvetic Diablerets nappe basal thrust | Large amounts of connate and metamorphic waters expelled upward, as indicated by 𝛿18O, 𝛿13C and 𝛿D in calcite or quartz veins, possibly overpressured by rapid tectonic burial[5] |
Sub-Alpine frontal thrust zone (Chartreuse and Vercors) and foreland (Valence basin) | pf measurements in exploration boreholes[6] | |
Pyrenees | Gavarnie thrust and splay faults | Fluid inclusions in quartz veins indicate fluctuations of pf between lithostatic (∼500 MPa) and hydrostatic (∼200 MPa)[7]; combination of shear reactivation and extension vein opening (𝜆 = 0.77–0.89)[8] |
Himalaya | Himalayan Main Central Thrust | Critical Coulomb wedge modeling suggests 𝜆 = 0.8–0.9[9], Coulomb stress change modeling suggests 𝜆 = 0.3–0.9[10] |
Apennines | Apenninic basal thrust zone, Apenninic foreland | Fluid inclusions in quartz veins indicate near-lithostatic pf at the time of the emplacement of the Liguride nappes[11]; calcite-cemented implosion breccias filling dilational jogs (pf > 𝜎3)[12] |
Taiwan | Taiwan fold-and-thrust belt basal decollement fault, Chelungpu fault | pf measurements in exploration boreholes (𝜆 ∼ 0.7)[13], critical Coulomb wedge modeling[14],[15]; discrete hydraulic fractures[16] |
Western California | Coast Ranges and western Great Valley | pf measurements in exploration boreholes[17],[18] |
References: [1]Burkhard et al. [1992], [2]Badertscher and Burkhard [2000], [3]Badertscher et al. [2002], [4]Hürzeler and Abart [2008], [5]Crespo-Blanc et al. [1995], [6]Deville [2021], [7]Henderson and McCaig [1996], [8]Lacroix et al. [2013], [9]Mugnier et al. [1994], [10]Cattin and Avouac [2000], [11]Mullis [1988], [12]Vannucchi et al. [2010], [13]Suppe and Wittke [1977], [14]Davis et al. [1983], [15]Barr and Dahlen [1990], [16]Boullier [2011], [17]Melchiorre et al. [1999], [18]Unruh et al. [1992].
The role of supra-hydrostatic pf build-up appears critical or ubiquitous in accretionary prisms, whatever active or inactive [Moore and Vrolijk 1992]. Table 2 provides examples of various continental margins or paleo-margins in which displacement along past or present (first-order) décollement faults or second-order faults (also referred to as splay faults) branching on décollements was or is possible due to supra-hydrostatic pf. Similarly to what is inferred along large-displacement thrusts, the supra-hydrostatic pf lowers
Examples of geological or geophysical evidence for supra-hydrostatic pore fluid pressures pf measured or inferred along low-angle reverse faults in accretionary prisms
Type of prism | Area | Type of structure | Evidence |
---|---|---|---|
Active accretionary prisms | Cascadia margin Oregon accretionary prism | Frontal décollement | Seismic velocity variations and negative polarity reflections in seismic profiles[1]–[3]; ODP core consolidation tests and logging data (𝜆 = 0.88–0.99)[4]; supra-hydrostatic pf (𝜆 = 0.8) computed from velocity-depth data[5]; reverse polarity reflections from the décollement[6] |
Central America Costa Rica and Guatemala margins | Frontal décollement | Direct measurement of pf in DSDP boreholes (𝜆 = 0.25–0.48)[7]; consolidation tests on ODP cores suggest excess pf (1.3–3.1 MPa)[8]; numerical modeling of pf build-up following sediment dewatering in undrained conditions[9]; pf measurements in ODP borehole hydrologic observatories (𝜆 = 0.25–1)[10]; pervasive veining suggests pf fluctuations in accordance with the seismic cycle[11] | |
SW Japan Nankai Trough accretionary prism | Frontal décollement | Shear tests on saturated clay samples from the décollement indicate that friction drops dramatically with increasing |
|
Splay fault | High-amplitude negative polarity reflections in seismic profiles[21],[22]; low impedance layer[23] | ||
Lesser Antilles Barbados Ridge accretionary prism | Frontal décollement | pf estimated from seismic velocity or from negative polarity reflections[24],[25]; direct measurements in ODP boreholes and sediment consolidation tests (𝜆 up to >0.9)[26],[27]; high pf suggested by anisotropy of magnetic susceptibility[28] | |
New Zealand Hikurangi accretionary prism | Pāpaku splay fault | Rock deformation experiments on cores from the fault zone (𝜆∗= 0.3–0.6)[29] | |
Exhumed inactive accretionary prisms | Shimanto accretionary prism (Japan) | Nobeoka paleo-décollement | Fluid inclusions and modeling suggest pf excess values between 5 and 20 MPa[30] |
Nakanomata splay fault | Quartz-cemented syntectonic dilatant hydraulic breccias[31] | ||
Kodiak accretionary prism (Alaska) | Paleo-décollements and mélange zones | Crack-seal quartz veins parallel to ancient decollements interpreted as resulting from repeated hydrofracturing (pf > 𝜎3)[32]; fluid inclusions indicate near-lithostatic pf (pf ∼𝜎1) at the time of quartz vein formation[33],[34]; lack of low-friction material and mechanical analysis of displacement along the décollement plead for supra-hydrostatic |
|
Chrystalls Beach accretionary prism (New Zealand) | Mudstone matrix of mélange | Shear veins severely misoriented with respect to stress field[38],[39] |
References: [1]Cochrane et al. [1994], [2]Cochrane et al. [1996], [3]Moore et al. [1995a], [4]Moore et al. [1995b], [5]Hyndman et al. [1993], [6]Tobin et al. [1994], [7]Von Huene [1985], [8]Saffer et al. [2000], [9]Screaton and Saffer [2005], [10]Davis and Villinger [2006], [11]Vannucchi and Leoni [2007], [12]Brown et al. [2003], [13]Ujiie et al. [2003], [14]Bangs et al. [2004], [15]Tsuji et al. [2008], [16]Skarbek and Saffer [2009], [17]Tobin and Saffer [2009], [18]Kitajima and Saffer [2012], [19]Hirose et al. [2021], [20]Pwavodi and Doan [2024], [21]Park et al. [2000], [22]Park et al. [2002], [23]Bangs et al. [1990], [24]Shipley et al. [1994], [25]Moore et al. [1995c], [26]Moore and Tobin [1997], [27]Moore et al. [1998], [28]Housen et al. [1996], [29]French and Morgan [2020], [30]Raimbourg et al. [2015], [31]Passelègue et al. [2014], [32]Fisher and Byrne [1987], [33]Vrolijk [1987], [34]Vrolijk et al. [1988], [35]Byrne and Fisher [1990], [36]Fisher et al. [1995], [37]Rowe et al. [2009], [38]Fagereng et al. [2010], [39]Fagereng et al. [2011].
ODP: Ocean Drilling Program. IODP: International Ocean Drilling Program. 3D: three-dimensional. pH: hydrostatic pressure. 𝜎m: mean stress (𝜎m = (𝜎1 + 𝜎2 + 𝜎3)∕3). 𝜆∗: modified pore fluid factor, 𝜆∗= (pf −pH)∕(pL −pH), where pH is the hydrostatic pressure and pL the lithostatic pressure caused by the weight of the overburden [Saffer and Tobin 2011].
3.2. Role of abnormally high pf in extensional tectonics
Large displacement along low-angle normal faults, also referred to as detachments, is mechanically paradoxical. The different mechanisms mentioned above to account for the displacement along misoriented fault surfaces apply to low-angle normal faults [Reynolds and Lister 1987; Yin 1989; Axen 1992; Westaway 1999; Abers 2009; Collettini 2011]. Table 3 provides examples of studies which suggest or demonstrate that reactivation of low-angle normal faults was possible because of supra-hydrostatic to lithostatic pf values. Regarding inactive detachments, structural evidence for high pf come from textures of precipitated minerals in breccia cements (so-called cockade structures) or in flat-lying crack-seal dilatant veins. Fluid inclusions in the precipitated minerals also provide indications. Lastly, mechanical modeling also accounts for overpressured-fluid-aided displacements along low-angle faults. Regarding active detachments, evidence consist of vein or breccia cement texture analyses (including fluid inclusions), mechanical modeling, pf measurements in boreholes, geophysical investigations (body wave velocity anomalies).
Examples of geological or geophysical evidence for displacement along low-angle normal faults (detachment faults) triggered or favored by supra-hydrostatic pore fluid pressures pf inside or near the fault zone
Type of low-angle normal fault | Name of structure, area | Evidence |
---|---|---|
Inactive intra-continental | Western US Basin and Range (Southern Mountains MCC deformed zones, Arizona; Whipple detachment, California; Siever Desert detachment, Utah) | Flat-lying dilatant veins suggest |
Central Alps (Brenner, Simplon and Grimsel faults) | Fluid inclusions in veins suggest supra-hydrostatic |
|
Gulf of Corinth LANF, Greece | Limit Analysis (maximum strength theorem analysis) suggests pf = 0.57–0.77 |
|
Active intra-continental | North Apennines LANFs, including Alto Tiberina and Tellaro detachments | Limit Analysis (maximum strength thorem analysis) suggests that pf = 0.57–0.77 |
Inactive intra-oceanic or intra-OCT | Detachment surfaces exposed in Alpine ophiolites | Calcite-cemented breccias (ophicalcites)[13],[14]; gouge veins injected in cataclasite suggest transient supra-hydrostatic |
Active intra-oceanic | Mid-Atlantic ridge Atlantis massif, mid-Atlantic ridge 13°–15° N | Fluid inclusions[16],[17]; hydraulic breccias[18] |
South Pacific Woodlark-D’Entrecasteaux ridge Moresby detachment | Low VP and high porosity values in the fault zone suggest supra-hydrostatic |
References: [1]Reynolds and Lister [1987], [2]Smith et al. [1991], [3]Selverstone et al. [2012], [4]Yuan et al. [2018], [5]Selverstone et al. [1995], [6]Axen et al. [1995], [7]Axen et al. [2001], [8]Berger and Herwegh [2019], [9]Yuan et al. [2020], [10]Collettini et al. [2008], [11]Clemenzi et al. [2015], [12]Moretti et al. [2009], [13]Früh-Green et al. [1990], [14]Picazo et al. [2013], [15]Manatschal [1999], [16]Escartin et al. [2003], [17]Castelain et al. [2014], [18]Picazo et al. [2012], [19]Floyd et al. [2001], [20]Roller et al. [2001], [21]Kopf et al. [2003], [22]Famin and Nakashima [2005].
MCC: metamorphic core complex. LANF: low-angle normal fault. OCT: ocean-continent transition. 𝜎v: principal vertical stress axis.
The other mechanisms listed above may also facilitate large displacement along low-angle normal faults. In particular, analog modeling suggests that extension can take place above continuous low-strength layers such as evaporites [Vendeville and Jackson 1992a, b]. Regarding the reorientation of the stress axes in the vicinity of the fault, Lecomte et al. [2011] propose to explain reactivation of misoriented low-angle normal faults by calling for an elasto-plastic frictional gouge instead of the classical frictional fault gouge. Plastic strain can initiate the displacement despite the misorientation. Following the initiation, stress axes near the fault zone rotate to a more favorable orientation with respect to the fault surface, allowing further displacement.
3.3. Role of abnormally high pf in strike-slip tectonics
The activity of steeply dipping to vertical plate-boundary transform or intra-plate strike-slip faults moderately to severely misoriented with respect to the principal stress axes constitutes a challenge since the discovery of nearlyperpendicular normal stresses acting along the San Andreas fault [Mount and Suppe 1987; Zoback et al. 1987]. As such, the San Andreas faults appears as a weak fault, and explanations for it have been extensively looked for. The models and mechanical analyses of Byerlee [1990, 1993] and Rice [1992] attempt to show that supra-hydrostatic pf could account for the weakness of the fault. Experimental measurement of clay permeabilities [e.g., Faulkner and Rutter 2001] provide a mechanism for the maintenance of excess pf in strike-slip fault zones. However, drilling across the fault zone during the SAFOD (San Andreas Fault Observatory at Depth) program at ∼3 km depth did not reveal any supra-hydrostatic pf, prompting researchers to propose other mechanisms that could explain the weakness: fault rocks with extremely low friction coefficients such as talc [Moore and Rymer 2007] or clays [Schleicher et al. 2009a, b; Carpenter et al. 2011; Holdsworth et al. 2011], stress axes reorientations to more favorable attitudes at short distances from the fault [Provost and Houston 2001; Hardebeck and Michael 2004; Healy 2008]. It seems clear that pressurized fluids do not weaken the San Andreas fault in its upper part (between the surface and a ∼3 km depth), except perhaps for micro-earthquake activity [Mittempergher et al. 2011]. Hydro-mechanical modeling [e.g., Fulton and Saffer 2009; Beeler et al. 2013] suggests that supra-hydrostatic pf could play a role in the weakening at depths larger than the SAFOD drilling. Conversely, hydro-mechanical modeling by Fulton et al. [2009] suggest that supra-hydrostatic pf cannot be maintained along the San Andreas fault at shallow (<3 km) depth, and that other mechanisms such as shear-enhanced compaction or thermal pressurization inside the fault zone have to be invoked.
Table 4 provides examples of transform or strike-slip faults along which supra-hydrostatic pf could play or could have played a role in the past or present fault activity. Direct evidence for abnormally high pf can be found in shallow boreholes drilled across the New Zealand Alpine fault, but no data from depths larger than a few hundred meters are available. Indirect evidence consist either in rare hydraulic fractures or in more frequently observed geophysical (electrical conductivity or seismic velocity) anomalies. In this last case, even if the anomalies can be interpreted as caused by the presence of fluids at depth, they do not bring undisputable evidence for pressurized fluids. The apparent scarcity of abnormally high pf ascertained along transform or strike-slip fault zones may be due to the lack or scarcity of efficient seals. Indeed, fault zones, especially their damage zones if any, crossing the entire crust may appear as conduits rather than barriers or seals.
Examples of geological or geophysical evidence for displacement along transform faults or strike-slip faults triggered or favored by supra-hydrostatic pore fluid pressures pf inside or near fault zones
Area, name of structure | Evidence |
---|---|
California, San Andreas fault | Very low resistivity, low VP, and high a VP∕VS ratio suggest supra-hydrostatic |
Turkey, North Anatolian Fault | Fluid inclusions in subhorizontal extension veins suggest supra-hydrostatic |
New Zealand, Alpine Fault | Slug tests in shallow (150 m deep) boreholes suggest that the near-surface Alpine fault zone may be site of high pf gradient[10]; pressure measurement during drilling reveals a slight (9%) supra-hydrostatic pf in shallow (∼900 m deep) borehole[11]; tremors appear to be located at 25–45 km depth, in a region of high P-wave attenuation that could be the site of supra-hydrostatic |
Japan, Atotsugawa fault | High pf could account for creep along some parts of the fault[13] |
Japan, Nojima fault | Discrete hydraulic fractures[14] |
Central Alps, Adamello pluton | Reactivation of the severely misoriented inactive Gole Larghe fault[15] |
Southeastern Brazil, Ribeira Shear Zone | Fluid inclusions in foliation-parallel or extensional quartz veins along the shear zone indicate pf fluctuations that are interpreted as the result of a fault-valve behavior[16] |
References: [1]Eberhart-Phillips and Michael [1993], [2]Mittempergher et al. [2011], [3]Hadizadeh et al. [2012], [4]Hadizadeh et al. [2024], [5]Janssen et al. [1997], [6]Pfister et al. [2000], [7]Karabulut et al. [2003], [8]Tank et al. [2005], [9]Karaş et al. [2020], [10]Sutherland et al. [2012], [11]Sutherland et al. [2017], [12]Wallace [2020], [13]Kato et al. [2007], [14]Boullier [2011], [15]Mittempergher et al. [2009], [16]Faleiros et al. [2007].
SAFOD: San Andreas Fault Observatory at Depth. DMT: diffusive mass transfert.
3.4. Conclusions
A review of the literature dealing with the role of abnormally high pf along faults in the brittle crust allows to draw the following key ideas.
(1) Undebatable evidence for supra-hydrostatic pf come from pressure measurements in oil industry or scientific boreholes (DSDP, OPD, IODP). Near-horizontal dilatant veins found along low-angle faults also constitute a strong evidence, especially when fluid inclusions preserved in these veins indicate supra-hydrostatic pf at the time of fluid entrapment. Geophysical anomalies, either electrical (conductivities) or seismological (seismic velocities), are interpreted as caused by abnormally high pf at depth along fault zones.
(2) Evidence, whatever direct or indirect, for supra-hydrostatic pf appear common along low-angle normal faults (detachments) or reverse faults (including décollements) (Tables 1–3). Fluids appear to be efficiently trapped beneath flat-lying or gently dipping allochtons that can act as impervious or low-permeability lids. Conversely, conclusive evidence for supra-hydrostatic pf along transform or strike-slip faults are scarcer than along low-angle normal or reverse faults (Table 4), possibly because such vertical or steeplydipping fault zones lack tight and laterally continuous seals that could prevent fluids from leaking upward to the surface.
(3) Along with fracturing and comminution linked with displacement along faults, fluid circulation and associated fluid-rock interactions quite often result in alteration of minerals, resulting in weaker minerals such as clays, talc and other minerals whose frictional properties are much weaker than primary minerals. This chemical effect of fluids is clearly a serious competitor with the pressurization of fluids to account for fault weakening.
(4) Mechanisms such as shear stress-enhanced compaction, thermal pressurization, or stress reorientation near fault zones certainly play roles in the reactivation of misoriented faults, but they are difficult to be recognized in the geological record.
4. Fluids and natural earthquakes
4.1. Intra-plate earthquakes, earthquake sequences, background seismicity, subduction earthquakes
Table 5 presents examples of studies that attempt to relate major intra-continental earthquakes, earthquake sequences, background seismicity, subduction earthquakes and aftershock sequences to abnormally high pf. Some of these examples were already introduced in the previous section, but without any specific mention to the seismogenic activity of the relevant faults.
Examples of geophysical evidence for major earthquakes and aftershocks triggered or favored by abnormally high pore fluid pressures pf in or around fault zones
Type of event | Area and/or events | Evidence |
---|---|---|
Intraplate earthquakes, earthquake sequences and background seismicity | Central Alps | Unexpected background seismic activity in the lower crust accounted for by supra-hydrostatic |
Himalaya MCT | Drop in VP and VS values, increase of |
|
Himalaya background sesimicity | Supra-hydrostatic pf at depth deduced from correlated seasonal variation of stress and seismicity[3] | |
Northern Apennines (1997–1998 Umbria-Marche-Colfiorito, 2000 Faenza and 2012 Emilia seismic sequences) | Supra-hydrostatic pf (𝜆⩾0.9) inferred from the presence at depth of Triassic evaporite impervious seals[4]; supra-hydrostatic pf (𝜆⩾0.7) suggested by stress inversion of focal mechanisms[5]; space–time evolution of seismic activity fits with diffusion laws, |
|
Central and southern Apennines (2009 Abruzzo-L’Aquila sequence, 2010–2014 Mt Pollino sequence) | Supra-hydrostatic CO2 pf values in exploratory boreholes[9],[10]; supra-hydrostatic pf propagation along a fault system tracked by space–time variations of VP∕VS anomalies during earthquake migration[11]; high VP∕VS and low QP∕QS indicative of supra-hydrostatic |
|
East Iceland rift zone | Stress inversion of focal mechanisms suggests near-lithostatic pf values (𝜆 ∼1)[17] | |
Back-arc side of NE Japan (M > 6 thrust-fault type events) | Mohr–Coulomb analysis suggests that severely misoriented faults are reactivated by supra-hydrostatic pf through a fault-valve mechanism[18] | |
Kaapvaal craton (2017 MW 6.5 Bostwana earthquake) | Episodic seismic activity in an otherwise stable craton explained by sporadic near-lithostatic pf (𝜆 ∼0.85)[19] | |
New Madrid seismic zone (1811–1812 sequence) | A test of the role of stress tensor shape 𝛷, fault friction coefficient 𝜇, pf, and gradient of 𝜎D with depth by a Mohr–Coulomb-based parametric analysis shows that reactivation of the faults requires supra-hydrostatic pf (𝜆 = 0.68–0.81), unless 𝜇 is less than 0.4[20] | |
Japan, Nojima fault, 1995 MW 7.2 Kobe earthquake | Seismic velocity anomalies near the hypocenter could reflect supra-hydrostatic pf that may have contributed to the initiation of the Kobe earthquake[21] | |
Cascadia | Seismic tomography indicates abnormal VP∕VS and Poisson ratio values interpreted as caused by near-lithostatic |
|
NE Japan | Aftershock focal mechanisms of the 1968 M 8.2 Tokachi earthquake suggest a weak plate interface accounted for by the post-seismic release of overpressured fluids following the main rupture[24]; seismic reflection imaging shows that the megathrust corresponds to a reflector with negative polarity, which could be explained by high pf having possibly triggered the the 2011 MW 9 Tohoku event[25]; mechanical analysis suggests stress permutations in the forearc region caused by fluctuations in pf during the seismic cycle (fault-valve action)[26] | |
SW Japan | 3-D seismic reflection data show the presence of a LVZ (∼2 km thick, ∼15 km wide, and ∼120 km long) beneath the Nankai accretionary prism, inside which inferred abnormally high pf may control interplate coupling[27] | |
Central America | Extremely high VP∕VS ratios in the entire subducting oceanic crust is interpreted as due to high pf. Varying velocity ratios in the overriding continental crust further allow to map varying coupling domains[28] | |
Chile | The importance of the post-seismic fluid discharge suggests overpressured pf beneath the subduction interface before large interplate events[29],[30]; 3-D seismic tomography allows to map the distribution, along the plate interface, of domains with abnormally high pf and domains with lower pf and to propose a correlation between these domains and the patches which slip aseismically (high pf) and those which are locked (low pf)[31] | |
New Zealand, Hikurangi subduction zone | A Mohr–Coulomb analysis suggests that the recurrence of megathrust earthquakes may be triggered by fluctuation of pf (from hydrostatic to lithostatic) in addition to stress accumulation[32] | |
Aftershocks | 1992 Landers earthquake | Increase of pf because of post-seismic fluid flow and Coulomb stress changes account for the chronology and spatial migration of the Landers event aftershocks[33] |
Apennines (1997 Umbria-Marche, 2009 L’Aquila, 2012 Emilia earthquake sequence) | Space–time migration of aftershocks fits with diffusion laws of fluids in porous media[34],[35]; inversion of earthquake nodal planes allows to map the 3-D pf distribution at depth in the hypocentral region of the L’Aquila earthquake and shows that time-space migration of aftershocks fits with diffusion laws of |
|
2011 Tohoku earthquake, NE Japan | Reactivation of misoriented fault planes of the aftershock seismic faults is accounted for by varying supra-hydrostatic |
|
2004 Sumatra earthquake | Aftershock migration along a splay fault merging with the megathrust is explained by upward migration of fluids, although supra-hydrostatic pf is not ascertained[40] |
References: [1]Deichmann [1992], [2]Mandal et al. [2022], [3]Bettinelli et al. [2008], [4]Quattrocchi [1999], [5]Boncio and Bracone [2009], [6]Lombardi et al. [2010], [7]Calderoni et al. [2009], [8]Pezzo et al. [2018], [9]Collettini et al. [2009a], [10]Chiodini et al. [2004], [11]Chiarabba et al. [2009a], [12]Chiarabba et al. [2009b]), [13]Di Luccio et al. [2010], [14]Lucente et al. [2010], [15]Chiodini et al. [2011], [16]Di Luccio et al. [2022], [17]Plateaux et al. [2012], [18]Sibson [2007, 2009], [19]Gardonio et al. [2018], [20]Leclère and Calais [2019], [21]Zhao et al. [1996], [22]Audet et al. [2009], [23]Peacock et al. [2011], [24]Magee and Zoback [1993], [25]Kimura et al. [2012], [26]Sibson [2013], [27]Park et al. [2010], [28]Audet and Schwartz [2013], [29]Husen and Kissling [2001], [30]Koerner et al. [2004], [31]Moreno et al. [2014], [32]Sibson and Rowland [2003], [33]Bosl and Nur [2002], [34]Miller et al. [2004], [35]Antonioli et al. [2005], [36]Terakawa et al. [2010], [37]Malagnini et al. [2012], [38]Pezzo et al. [2018], [39]Leclère and Fabbri [2013], [40]Waldhauser et al. [2012].
MCT: Main Central Thrust. QP∕QS: attenuation ratios of P and S waves. 3-D: three-dimensional. LVZ: low seismic velocity zone. 𝛷: stress tensor shape; 𝛷 = (𝜎2 −𝜎3)∕(𝜎1 −𝜎3).
Except rare examples of direct measurements in boreholes, evidence for supra-hydrostatic pf triggering or favoring intra-plate earthquakes are mostly indirect. Body-wave velocity anomalies detected by two-dimensional or three-dimensional tomography suggest that pressurized fluids are present in hypocentral regions of many earthquakes. Most authors agree that fluids are trapped at depth and that their accumulation results in abnormally high pf values which in turn weaken the faults.
Evidence for abnormally high pf that could either trigger or favor the propagation of subduction zone earthquakes mainly comes from body wave velocity anomalies revealed by tomography (Table 5). Other indirect evidence come from mechanical analyses based on the reactivation of misoriented fault planes [e.g., Sibson 2013]. The analysis of Seno [2009], based on the construction of the differential stress profiles across several subduction zones, shows that the pore fluid pressure ratio 𝜆 accounts for much of the interplate coupling.
4.2. Aftershocks
4.2.1. Present-day aftershocks
In many cases, aftershocks following large magnitude events typically migrate both in space and time. This was first noticed by Nur and Booker [1972] following the fortuitous or induced seismic sequences at Denver and Rangely [Healy et al. 1968; Raleigh et al. 1976]. Since then, precise hypocenter relocations show that the temporal and spatial migration follows laws of diffusion of pressure fronts in porous media, suggesting that pressurized fluids contribute to trigger aftershocks (Table 5). The theoretical analysis of Miller [2020], based on the modeling of permeability fluctuations with time during the seismic cycle, further shows that major events not characterized by co-seismic or post-seismic releases of large amounts of fluids are followed by very few aftershocks, whereas those characterized by the release of significant amounts of co- or post-seismic fluids will be followed by large aftershock populations. Even if other mechanisms such as loading of faults by Coulomb stress transfer can influence the triggering or the propagation of aftershocks [Nostro et al. 2005], the role of supra-hydrostatic pf appears significant in many cases.
4.2.2. Aftershocks in the geological record
Because of their small magnitudes, aftershocks related to past earthquakes are uneasy to recognize in the geological record. However, a specific type of aftershocks, called “golden” aftershocks, is presumably recognized in Archean rocks (Yilgarn craton, western Australia) where mechanical analyses based on Coulomb stress transfer following major earthquakes suggest that transiently abnormally high pf triggered ruptures on secondary faults after main events [Cox and Ruming 2004; Micklethwaite 2008]. Indeed, the Yilgarn craton gold deposits hosted in secondary low-displacement hectometric faults and shear zones do not show a random spatial distribution, but are clustered in rock volumes near terminations of primary plurikilometric strike-slip fault segments. Mechanical analyses of the stress transfer following major earthquakes along any of the fault segments show that specific rock volumes are stress-loaded ahead of the segment terminations [so-called “loaded” lobes, inside which the stress state acting on pre-existing fractures makes them close to failure; King et al. 1994]. Pressurized fluid expelled from the main shock hypocentral volume then percolates through the fracture network in the loaded lobes, triggering eventual ruptures and allowing, by pressure drop, deposition of gold-bearing mineralization. The repetition of main shocks and aftershocks eventually results in deposits of economical interest.
4.3. Subducting slab intermediate-depth earthquakes
4.3.1. Seismological evidence for supra-hydrostatic pf in currently subducting slabs
In subduction zones, intermediate-depth earthquakes, that is, earthquakes with hypocentral depths between ∼50 and 300 km, are no longer localized along the plate interface, but inside the subducting slab. Various mechanisms have been proposed to account for nucleation or propagation of this intermediate-depth seismicity [Frohlich 2006; Houston 2015]: (1) dehydration embrittlement, in which locally abnormally high pf allows a transition from a viscous regime of deformation to brittle faulting, (2) thermal instabilities, or (self-localizing) thermal runaway, in which incipient ductile shear zones develop as rheological instabilities along which brittle failure can nucleate; (3) “anticrack faulting”, triggered by mineral phase change reactions. Among these mechanisms, which can act in concert, the first one is of peculiar interest here. As summarized in Table 6, evidence or suspicion for supra-hydrostatic pf come from three-dimensional seismic tomography across subducting slabs currently in their post-seismic stage or in their inter-seismic stage.
Examples of geological and geophysical evidence for intermediate-depth seismicity in modern or fossil subduction zones triggered or favored by supra-hydrostatic pore fluid pressures pf
Setting | Area | Name of structure | Evidence |
---|---|---|---|
NE Japan | Japan trench (subducting Pacific slab) | Seismic tomography indicates abnormal VP∕VS and Poisson ratio values interpreted as caused by near-lithostatic |
|
Central Andean subduction | Chile trench (subducting Nazca plate) | High pf could have contributed to the nucleation or to the propagation of a MW 7.8 earthquake in northern Chile at a 115 km depth[6]; high VP∕VS ratios in the subducting Nazca plate and thermodynamic modeling of dehydration reactions suggest high pf at depths >50 km[7] | |
Lesser Antilles | Subducting Atlantic plate | Hypocenters between 120 and 160 km are distributed where seismic tomography indicates low VP values and high VP∕VS ratios, suggesting high |
|
Middle America trench | Middle America trench (subducting Cocos plate) | Nucleation of a MW 7.1 earthquake at a 57 km depth in the subducting Cocos plate tentatively explained by high pf resulting from the brucite + antigorite = olivine + H2O reaction[9] | |
Fossil subduction zones | Central Alps | Dent Blanche basal thrust | Cross-cutting relationships between foliated cataclasites, mylonites and quartz-clinozoisite veins (0.95 <𝜆< 1)[10] |
Valpelline Shear Zone between Valpelline and Arolla units, Dent Blanche | Metamorphic tensile veins (𝜆 ∼ 0.7)[11] | ||
Western Alps | Monviso | HP crack-seal veins in eclogite-facies metagabbros and oscillatory growth zoning of minerals as products of precipitation suggest fluctuations of |
|
Lanzo | Hydrofracturing of omphacitites by CH4–H2-rich fluids at 30–80 km depth[15] | ||
California | Franciscan Complex | Rhythmic chemical zoning in HP garnets result from growth-dissolution cycles driven by pressure pulses (pf = 100–350 MPa)[16] | |
Zambia | Relics of subducted lower oceanic crust, late Precambrian Zambezi belt | Hydraulic fracturing[17] |
References: [1]Nakajima et al. [2001], [2]Mishra and Zhao [2004], [3]Nakajima et al. [2011], [4]Shiina et al. [2013], [5]Shiina et al. [2017], [6]Kuge et al. [2010], [7]Bloch et al. [2018], [8]Paulatto et al. [2017], [9]Gutiérrez-Aguilar et al. [2022], [10]Angiboust et al. [2015], [11]Menant et al. [2018], [12]Spandler et al. [2011], [13]Angiboust and Raimondo [2022], [14]Hoover et al. [2022], [15]Giuntoli et al. [2024], [16]Viete et al. [2018], [17]John and Schenk [2006].
HP: high pressure metamorphic conditions.
4.3.2. Evidence for excess pf at intermediate depths in the geological record
Table 6 further provides examples of structures (crack-seal veins, mineral overgrowths) reported from high-pressure metamorphic terranes which can be interpreted as resulting from overpressured fluids during intermediate-depth seismic activity. Brittle dilatant veins crossing ductilely deformed high-pressure rocks are one convincing piece of evidence for abnormally high pf. Similarly, finely zoned rims around garnets in eclogitic rocks can be interpreted as repeated growths of thin garnet layers following cyclical arrivals of co-seismic fluids. In these cases, the sharpness of the boundaries between the domains with distinct element concentrations are sharp, whereas they should be irregular or diffuse in the case of intracystalline high-temperature solid diffusion, confirming the fluid pulse hypothesis. Through a detailed analysis of such zoned garnet overgrowths, Viete et al. [2018] estimate that at least four overpressured fluid pulses occurred in less than 300,000 years.
4.4. Seismic swarms
Whatever they are related to volcanic systems or not, many seismic swarms appear to be triggered or favored by pressurized fluids (Table 7). The most convincing argument for the role of pf lies in the fact that, like for aftershock sequences, the spatial and temporal migration of hypocentres follows diffusion laws of pressure front propagation in porous media. Several authors however propose that other mechanisms can also play a role, in combination with pf wave propagation. A frequently invoked additional mechanism is Coulomb stress transfer [Aoyama et al. 2002; Hainzl 2004; Yukutake et al. 2011; Fischer et al. 2014]. In particular, studying a swarm developed near the Hakone volcano, central Japan, Yukutake et al. [2011] were able to show that the hypocenters of a first earthquake sequence were aligned along fault-like planar surfaces, while those of a subsequent sequence were located in compressive lobes associated with the planar surfaces, demonstrating the sequence fluid-triggered events followed by Coulomb stress transfer-triggered events. Besides, Aoyama et al. [2002] call for stress corrosion as an additional mechanism in the triggering of seismic swarms.
Examples of geophysical evidence for seismic swarms or seismic swarm-like sequences triggered or favored by abnormally high pore fluid pressures pf in or around fault zones
Area and/or events | Evidence |
---|---|
Central and northern Japan (Matsushiro, Tohoku, Hida mountains, Hakone volcano) | Space–time evolution of seismicity fits with pf diffusion laws[1],[2],[3]; pressurized fluid diffusion accounts for space–time evolution of seismicity along with Coulomb stress transfert and stress corrosion[4]; pressurized fluid diffusion and Coulomb stress transfert account for space–time evolution of seismicity[5] |
Dobi graben seismic sequence, Afar rift, Djibouti | Space–time evolution of seismicity fits with pf diffusion laws[6] |
East African Rift, Uganda, Rwenzori region | Spatial overlap in the middle crust between intense volatile and CO2 circulation and swarm hypocentral regions[7] |
Central Europe (West Bohemia/Vogtland), | Space–time evolution of seismicity fits with pf diffusion laws[8],[9], but static and dynamic Coulomb stress changes also play a role[10],[11],[12]; at about 9 km depth, 𝜆 ∼ 0.98 (pf = 244 MPa, that is 5 MPa below 𝜎v)[13] |
Western Europe (Vosges mountains) | Space–time evolution of seismicity fits with pf diffusion laws[14] |
Western Alps (Ubaye region and vicinity) | Space–time evolution of seismicity fits with pf diffusion laws[15],[16][17]; supra-hydrostatic pf are necessary for reactivation of misoriented faults (𝜆 = 0.41–0.51)[18]; time-space evolution of seismicity and development of excess pf (between 35 and 55 MPa) explained by creep compaction[19]; space–time evolution of seismicity fits with pf diffusion laws, but co-seismic stress transfer explains the seismicity close to the mainshock source[20] |
Corinth rift | Space–time evolution of seismicity fits with pf diffusion laws[21],[22] |
Southern Italy, Mt Pollino region | Space–time evolution of seismicity fits with pf diffusion laws[23] |
Southern California (including Long Valley caldera) | Space–time evolution of seismicity fits with pf diffusion laws[24],[25], possibly combined with episodic aseismic slip[26] |
References: [1]Nur [1974], [2]Cappa et al. [2009], [3]Yoshida et al. [2016], [4]Aoyama et al. [2002], [5]Yukutake et al. [2011], [6]Noir et al. [1997], [7]Lindenfeld et al. [2012], [8]Parotidis et al. [2003], [9]Hainzl [2004], [10]Hainzl and Fischer [2002], [11]Fischer and Horálek [2005], [12]Fischer et al. [2014], [13]Vavryčuk [2002], [14]Audin et al. [2002], [15]Jenatton et al. [2007], [16]Godano et al. [2013], [17]Baques et al. [2021], [18]Leclère et al. [2012], [19]Leclère et al. [2013], [20]De Barros et al. [2019], [21]Duverger et al. [2015], [22]De Barros et al. [2020], [23]De Matteis et al. [2021], [24]Prejean et al. [2003], [25]Shelly et al. [2016], [26]Vidale and Shearer [2006].
4.5. Fluid overpressures and slow earthquakes
Slow earthquakes, a collective name including slow-slip events, low- or very-low-frequency earthquakes, low-frequency tremors, non-volcanic tremors, and episodic tremor-and-slip events, are in many cases suspected to be triggered or favored by supra-hydrostatic pf. Table 8 is a non-exhaustive compilation of studies that relate slow earthquakes (in the broad sense) with evidence for supra-hydrostatic pf. With the exception of one study pertaining to the San Andreas fault, all other studies deal with slow earthquakes in subduction zones. Direct evidence come from measurements in ODP or IODP boreholes equipped with pressure or fluid flow sensors [Brown et al. 2005; Araki et al. 2017]. Indirect evidence are provided by geophysical methods (seismic tomography or magneto-tellurics) that show that domains characterized by seismic velocity anomalies or by low resistivities, which are explained by the presence of possibly overpressured fluids, overlap zones of nucleation of slow events. Besides, laboratory experiments carried out on oceanic crust rocks (gabbros, serpentinites) and numerical modeling further support the interplay between supra-hydrostatic pf and slow earthquakes [Peacock 2009; Katayama et al. 2012; Kitajima and Saffer 2012; Beeler et al. 2013; Saffer and Wallace 2015; Bernaudin and Gueydan 2018; Condit et al. 2020; Dal Zilio and Gerya 2022; Eberhard et al. 2022].
Examples of geological or geophysical evidence for present-day or past slow earthquakes triggered or favored by abnormally high pore fluid pressures pf in or around fault zones
Setting | Area, structure, unit | Evidence |
---|---|---|
Present-day events along plate interfaces, subduction zones | Cascadia | Seismic imaging and seismic wave analyses suggest that pf is near-lithostatic in a LVL inside which SSEs or ETSs nucleate[1],[2],[3],[4]; supra-hydrostatic pf inferred in anisotropically permeable foliation parallel to the plate interface explains NVT distribution[5]; 3-D seismic tomography and magneto-tellurics show that LFEs nucleate in a landward dipping region characterized by a high Poisson ratio and by a high electrical conductivity; the region is interpreted as saturated by fluids trapped at near-lithostatic |
Middle America trench | Episodic fluid flow pulses measured in ODP boreholes correlate with seismic tremor in the frontal part of the plate interface and could reflect expulsion of transiently overpressured fluids[8]; SSEs nucleate in an ultra-slow velocity layer inside which fluids may be overpressured[9]; LFE occurrence during a SSE is interpreted to result from a pf fluctuation having migrated updip along the subduction interface[10] | |
NE Japan (Japan trench) | In the upper part of the downgoing Pacific, between 60 and 80 km depth, a zone of high VP∕VS is accounted for by high |
|
SW Japan (Nankai subduction zone) | NVDT possibly linked with high |
|
New Zealand (Hikurangi margin) | Deep SSEs occur in a zone characterized by a high P wave attenuation and by a high VP∕VS ratio, suggesting high |
|
Present-day events along plate boundary transform faults | San Andreas fault | NVT activity near Parfield following large earthquakes seem to be enhanced by static shear stress and Coulomb stress increases, but pf fluctuations at depth (15–30 km) may also play a role[27]; NVT near Parkfield likely reflect shear failure on a critically stressed fault in the presence of near-lithostatic |
Evidence in the geological record along subduction zone plate interfaces | Namibia (Damara belt) | Hydrothermal quartz veins preserved in ductilely deformed rocks attest for hydrofracturing caused by high pf during LFEs and VLFEs in the plastic aseismic regime below the seismogenic zone[29] |
Cyclades (Cycladic blueschist unit, Syros) | Brittle deformation structures (shear fractures and veins) in eclogite lenses embedded in ductilely deformed blueschist are interpreted to result from SSEs triggered by high |
|
SW Japan (Triassic Tomuru blueschists) | Quartz-filled crack-seal shear and extension veins in subduction mélange formed by frictional sliding and tensile fracturing at near-lithostatic pf at short reccurrence times (a few years, determined from kinetic modeling of quartz precipitation) are tentatively accounted for by episodic strain release during ETSs[31] | |
New Zealand (Dun ophiolite, Livingstone Fault) | Field and microstructural observations suggest that chemical reactions involving serpentinite can promote rock hardening and generate in situ fluid over-pressure patches and brittle failure in the source region of deep tremor along the slab-mantle interface[32] | |
Alaska (Kodiak accretionary complex) | Quartz vein microstructures and estimation of duration of local silica diffusion indicate that near-lithostatic pf can produce crack seal bands in less than 10 days, which is similar to the duration of slow earthquakes[33] | |
Northern Apennines (continental metasediments) | Syn-mylonitization quartz-carpholite dilational shear veins formed under HP metamorphic conditions as a result of high pf are interpreted as a record of deep ETS events[34] | |
Central Alps (Arosa zone) | Crack-seal veins formed under blueschist facies conditions concurrently with viscous flow as a result of near-lithostatic pf; the low estimated values of differential stresses suggest that the veins formed during slow slip and tremor events[35] | |
California (Franciscan complex eastern belt) | Opening increments of ∼1 mm in blueschist-facies metasediments are interpreted as the result of transient near-lithostatic pf during LFEs[36] |
References: [1]Abers et al. [2009], [2]Audet et al. [2009], [3]Tauzin et al. [2017], [4]Audet and Schaeffer [2018], [5]Delph et al. [2018], [6]Calvert et al. [2020], [7]Gosselin et al. [2020], [8]Brown et al. [2005], [9]Song et al. [2009], [10]Frank et al. [2015], [11]Shelly et al. [2006b], [12]Obara [2002], [13]Seno and Yamasaki [2003], [14]Kodaira et al. [2004], [15]Shelly et al. [2006a], [16]Matsubara et al. [2009], [17]Kato et al. [2010], [18]Sugioka et al. [2012], [19]Araki et al. [2017], [20]Ariyoshi et al. [2021], [21]Wallace and Eberhart-Phillips [2013], [22]Bassett et al. [2014], [23]Eberhart-Phillips and Bannister [2015], [24]Shaddox and Schwartz [2019], [25]Warren-Smith et al. [2019], [26]Yarce et al. [2021], [27]Nadeau and Guilhem [2009], [28]Thomas et al. [2009], [29]Fagereng et al. [2018], [30]Behr et al. [2018], [31]Ujiie et al. [2018], [32]Tarling et al. [2019], [33]Fisher and Brantley [2014], [34]Giuntoli and Viola [2021], [35]Condit and French [2022], [36]Schmidt and Platt [2022].
LVL: low-velocity layer. SSE: slow-slip event. ETS: episodic tremor and slip. NVT: non-volcanic tremor. 3-D: three-dimensional. LFE: low-frequency earthquake. NVDT: non-volcanic deep tremor. ODP: Ocean Drilling Program. LFT: low-frequency tremor. OBS: ocean-bottom seismometer. VLFE: very low frequency earthquake. HP: high pressure.
4.5.1. Evidence in the geological record
Tracking slow earthquake phenomena in the geological record is not easy, since rocks only exhibit frozen structures, for which strain rates are difficult to estimate. However, several studies contend that past slow slip events left tracks in surface-exhumed fault rocks (Table 8). Most of such studies pertain to ancient subduction interface-related slow events triggered or facilitated by near-lithostatic pf. These studies are based on scenarii that are compatible with cyclic fracturing and fluid arrival in the fractured zones. One key issue in the slow-earthquake interpretation is the estimated duration of events. Based on silica diffusion from wall rock to quartz crak-seal veins found along a shear zone adjacent to a paleo-décollement in the Kodiak accretionary complex, Fisher and Brantley [2014] could estimate the time necessary to precipitate quartz for one crak-seal band as less than 10 days, which could correspond to the duration of some slow events in subduction zones. Similarly, based on a kinematic model of the growth of microscopic quartz bands following cyclical pore fluid ingression in crack-seal veins from the Shimanto accretionary prism, Ujiie et al. [2018] could estimate the minimum duration of precipitation between two successive fluid pulses as less than 5 years. Such a duration is too low for standard earthquake rupture, but could correspond to episodic tremor and slip. Overall, the recognition of slow earthquakes in the geological record is still speculative, but quantification of duration of various inter-seismic or co-seismic phenomena such as mineral precipitation could validate the inferred geological signature of slow events.
4.6. Fluids and man-made earthquakes
Several man-made earthquakes show that fluids can trigger seismic ruptures. One emblematic piece of evidence for this triggering role is the earthquake activity that occurred in Colorado following waste water disposal at depth [Healy et al. 1968]. Experimental water injection at depth at Rangely [Colorado, Raleigh et al. 1976] confirmed than fluids could trigger earthquakes. Since then, the artifical triggering of earthquakes has been ascertained in a large variety of human activities: hydrocarbon extraction [Wiprut and Zoback 2000; Amos et al. 2014; Bourne et al. 2014; Van Wees et al. 2014], dam reservoir impoundment [Rastogi et al. 1997; Gupta 2002; McGarr et al. 2002; Zhang et al. 2019], industrial waste water repository [Horton 2012; Yeck et al. 2016], carbon dioxide storage projects [Zoback and Gorelick 2012], and water injection in deep boreholes to produce geothermal energy [Deichmann and Giardini 2009; Terakawa et al. 2012; McGarr et al. 2015]. Fluid-injection-experiments at the KTB borehole in Germany have shown that even small pressure variations (<1 MPa) could trigger numerous microearthquakes at a depth of 9 km [Zoback and Harjes 1997]. In most case studies, the pf build-up in the vicinity of critically loaded faults is responsible for failure. However, abnormally high pf may also act indirectly by triggering aseismic slip which in turn leads to an earthquake [Wei et al. 2015]. Lastly, stress perturbations by Coulomb stress transfer can also contribute to man-induced seismic ruptures [De Matteis et al. 2024].
4.7. Conclusions
The interactions between fluids and seismic ruptures in the brittle crust or in subducting slabs are more and more recognized or suspected (Tables 5–8). The role of overpressured fluids in the triggering or propagation of small-magnitude events such as aftershocks, swarms and, to a lesser extent, slow earthquakes, seems to be well established. Regarding large-magnitude events, the role of excess pore fluid pressures is more difficult to ascertain. However, a large body of evidence suggests that seismic ruptures, especially those along plate interfaces in subduction zones, take place in crustal volumes where seismic tomography indicates body wave velocity anomalies that are interpreted as the consequence of the presence of overpressured fluids. The present review of literature further shows that abnormally high pore fluid pressures may not be the only mechanism. Indeed, stress perturbations can act in concert with abnormally high pf and the inferred decrease of 𝜎n.
5. Conclusions
A review of the literature shows that fluids physically interact everywhere and at all scales with deformation in the brittle crust and in subducting slabs. Since they are more permeable than most intact rocks, fractures, faults and shear zones in the brittle or brittle-ductile crust and in the subducting slabs appear as efficient paths for fluid circulation. Provided that impervious seals develop transiently or permanently, fluids can accumulate and their pressure can increase. Where faults are critically stressed, only a minor overpressure value (a few MPa above the hydrostatic pressure) can trigger or favor rupture or displacement. Where faults are not critically stressed, for instance where they are severely misoriented with respect to the active stress tensor, larger excess pf values are needed for reactivation. Fluid overpressures can be estimated by using chemical data or fluid inclusion data in exhumed rocks. Estimated values are as follows: 25–135 MPa at seismogenic depths [ca. 5 to 10 km, Shimanto paleo-accretionary prism, Raimbourg et al. 2022, Kodiak paleo-accretionary prism, Vrolijk 1987], 50–150 MPa at 8–9 km [Apennines, Mullis 1988], 110 MPa at ca. 20 km [Central Alps, Berger and Herwegh 2019], 200–350 MPa at 8–10 km or deeper [Val d’Or district, Canada, Robert et al. 1995], 100–350 MPa at 30–70 km in an exhumed subducted lithosphere [Viete et al. 2018].
The influence of abnormally high pf is of utmost importance in the triggering or in the propagation of seismic ruptures. The pf effect appears to be common in small to moderate magnitude (M < 5 ∼ 6) events such as aftershocks or swarm sequences. The role of high pf is less obvious for large magnitude (M > 6 or higher) events. However, the precursor mechanisms preceding large magnitude events may be partly controlled by pf build-up. Future researches will concentrate on this aspect of the seismic cycle. In particular, a critical issue is to try to determine whether a pf increase is the triggering (causal) mechanism for a seismic rupture propagation or is a consequence of a propagating rupture triggered by a mechanism that would not be related to pf. In their spatio-temporal monitoring of VP and VS variations during the 1997 Umbria-Marche sequence, central Italy (Table 5), Chiarabba et al. [2009a] suggest that the pf propagation precedes the seismic ruptures. A similar conclusion is reached by Yoshida et al. [2023] in their study of a swarm sequence resulting in a Mw 6.2 event in NE Japan. Besides, the in-situ experiments of Guglielmi et al. [2015] and Cappa et al. [2022] show that a pf increase triggers aseismic slip that eventually turns into a seismic displacement. From their field study of intermediate-depth earthquakes frozen in an ancient oceanic crust, John and Schenk [2006] conclude that seismic rupture was not preceded but rather accompanied and followed by fluid ingression in the hypocentral volume (Table 6). Further experimental or in situ works along with detailed geological or geophysical analyses are obviously needed before the complex interrelationships between pf variation and inception or propagation of brittle fracture or slip along faults can be fully understood.
High pf values also play a role in the triggering or maintenance of slow slip earthquakes and related phenomena along several subduction interfaces or along transform faults. Since these transient phenomena can in turn influence or control large-magnitude seismic ruptures, a careful analysis or monitoring of pf build-up is an important direction of future research. The precursory results of Brown et al. [2005], Mikada et al. [2006], Fulton and Brodsky [2016] or Ariyoshi et al. [2021] are examples of such a promising monitoring.
Declaration of interests
The authors do not work for, advise, own shares in, or receive funds from any organization that could benefit from this article, and have declared no affiliations other than their research organizations.