1 Introduction
This article examines the origin, occurrence, and role of chance in biological systems, at all levels of organization, from genes to ecosystems and the biosphere, and at all related scales of time and space. Chance is largely responsible for the processes behind spontaneous biological diversification, including its emergence and growth as well as continuity and even decline. The mechanisms behind chance belong to two categories, including those outside of biological systems, and thus belonging to their environment (such as electromagnetic radiations), and those that are a part of these systems. We will focus on this latter category of mechanisms. We call them ‘biological roulettes’ because, like a roulette wheel, the results they produce seem random.1 In keeping with the same analogy, we assume that they are complex, deterministic systems (i.e., a set of biochemical reactions or ecological interactions) functioning in chaotic domains. Precisely, in a previous paper, we have discussed how chaotic dynamics, produced by a deterministic system, can behave like pseudo-random sequences of events [1]. Therefore, it is reasonable to assume that such mechanisms also play a role in the functioning of life systems. They offer one fundamental advantage: a kind of ‘life insurance’ in the face of unpredictable environmental hazards. This is the principal topic of this paper.
The debate over chance and necessity is not new and concerns many disciplines [2]. For instance, the role of chance in evolutionary processes and heredity was identified early in the well-known studies conducted by Darwin and Mendel. However, the first modern synthesis was developed by Jacques Monod [3]. He considered both what might be affected by chance, mainly genetics, and its importance, particularly in evolution, as well as the need for organisms to survive and remain healthy, to adapt themselves to their environment and, in this way, have selective advantages.
Briefly, random events produce variations in the structure of the genome (mutations), but only some of them lead to viable organisms: this is known as ‘functional necessity’. Few of these organisms and their offspring elude natural constraints: this is known as ‘selective necessity’.
In his fundamental book on the logics of life, François Jacob discussed how biological diversity is produced in particular by random processes occurring during sexual reproduction [4]. Jacob denoted the role of diversification processes, which could lead to the emergence of different organisms more or less adapted to resisting environmental hazards. These processes act much as a ‘life insurance’ for species, but also for life itself in the event of major disasters, such as has occurred throughout the history of the planet.
Many other authors have participated in this debate or borrowed from these concepts, including McCann [5] and Carroll [6]. Carlton and Geller [7] described another kind of chance that they called ‘ecological roulettes’: the transportation of marine species far from their original ecosystem either by natural means such as ocean currents or by man-made devices such as boats. If they are already adapted to these new sites, they can colonize them and, in this way, help to spread their populations. The result is something that seems random, but the analogy with the roulette stops there.
2 Where does chance come from?
Classically, chance is considered to be either the result of external, uncontrollable events (such as the electromagnetic radiations that cause mutations; the turbulence, currents or maritime routes that result in the displacement of marine species), or due to imperfect mechanisms within biological or ecological systems. Those that trigger chromosomal crossing-over or the dispersal of plant seeds in an ecosystem are examples of such mechanisms. Many other well-known biological processes rely on chance, including, inter alia, chromosomal migrations during cell mitosis, and the transfer of gametes during fertilization. Finally, based on recent results, for example on the interaction between environmental conditions and genome expression and transmission, we may assume that certain biological roulettes could be also influenced by environmental events or the general environmental context (see the example of epigenetics below).
However, it is noteworthy that these ‘imperfect’ mechanisms are still present after a very long period of evolution, whereas many other mechanisms are extraordinarily clever and precise from a deterministic point of view. This is the case, for example, of physiological regulatory mechanisms in organisms, the regulations in metabolic pathways, or maternal behaviour in higher species. So, can we consider the persistence of a stochastic mechanism as a by-product of natural selection? Or is it a major consequence, because chance provides living systems with certain advantages? And, finally, were the mechanisms that produce random processes preserved or even selected during evolution?
We truly can compare these mechanisms to a mechanical roulette in the sense that they are biological or ecological ‘devices’ producing outcomes that look like random events. In other words: do biological or ecological roulettes exist and have they been selected to produce events that look random?
3 Stochasticity occurs everywhere in living systems
Stochasticity is present at many levels in the hierarchical organization of living systems, from the genome to ecosystems (Table 1).
Examples of mechanisms producing results with important, even essential, components, but that seem like the result of random processes. Inner biological mechanisms are much more numerous than outer ones coming from the environment (electromagnetic radiation; chemical compounds, such as mutagens; fluid turbulences for pollens or seed dispersal). Like the mechanical devices in a casino, biological roulettes may be deterministic mechanisms functioning in a chaotic domain
Biological level of organization | Biological processes that may exhibit important random components |
Gene | Local mutations: addition, deletion, change of a nucleotide. |
Local modification: e.g., methylation of bases. | |
Genome | Deletion, insertion, transposition of DNA sequences in a genome, duplication of genes… |
Organism | Gene transfers (e.g., plasmides in bacteria). |
Reproduction: Gamete production (mechanisms of gene exchange: crossing-over, migration of chromosomes): production of a large diversity of gametes from the same genome structure. | |
Immunology: production of a large diversity of potential antibodies. | |
Gene expression: random expression of a gene or of a set of genes, role of epigenetic mechanisms. | |
… | |
Population | Reproduction mechanisms, particularly of sexed organisms (choice of partner, direct or indirect fertilization*): production of a diversity of offspring within the species to which this population belong. |
Hybridization: gene transfer between neighbouring species. | |
Migration, casual isolation of groups of individuals. | |
(*) Direct fertilization, mainly in animals (copulation), indirect fertilization, mainly in plants (e.g., aerial or animal dissemination of pollens). | |
Community and ecosystem | Cooperation between species, e.g., between plants and animals for seed and pollen dissemination. |
Mutations take place in the genome, for example, and modifications in the genome's structure can be the result of the insertion, deletion, or transfer of pieces of DNA as well as the duplication of genes. Transposons, when they move, also modify gene sequences in genomes. Eventually, reversible local modifications, such as the methylation of a base in the DNA sequence or the synthesis of interferent microRNA from specific DNA regions, can change the expression of a gene from one generation to the next and could be important evolutionary factors. These are epigenetic means of transmission, which disturb the classical molecular interpretation of the Mendelian scheme. They are also sensitive to environmental conditions. We can cite examples of the ICF (Immunodeficiency, Centromeric instability, Facial anomalies) syndrome [8], the kit gene of the mouse [9] and HAR 1 (Human Accelerated Region gene [10]); the latter is what seems to distinguish clearly man from chimpanzee. We should to point out that the Nobel Prize has been awarded to Craig Mello and Andrew Fire for their work on genetic regulations in the nematode worm Caenorhabditis elegans, in which epigenetic processes (precisely RNA interference) are involved [11]. An overview on epigenetics can be found in [12] and [13]. It is reasonable to assume that some biological roulettes play a role on the structures or processes involved or, at least, the corresponding parts of DNA are subject to the same changes as those listed above. This set of processes and their possible interactions with environmental factors, which are a source of biological diversity, still remain largely unknown and represent an exciting avenue for research.
In organisms, antibody proteins are continuously synthesized from a limited number of genes, but with particular arrangements to produce mRNA from DNA (which looks like combinatory processes), and then the random production of protein sequences. As the structure of an infectious agent is a priori unpredictable, one solution is to produce ‘randomly and continuously’ a great diversity of such proteins [14]. Some bacterial genes may also be expressed randomly [15]. For sexed organisms, during the meiotic phase when gametes are produced, genes are transferred between chromosomes (this is known as ‘crossing-over’) that make the genetic structure of the gametes produced different from those of the parent organism.
In populations, even human populations, the choice of a partner for sexual reproduction is, at least in part, random, and is yet another source of biological diversity in the offspring.
In ecosystems, natural systems are generally diversified. The individuals of a great number of species – trees, for example – are distributed quasi-randomly in space. Moreover, the scale of heterogeneity can be small (e.g., a group of few individuals). Random processes are largely responsible for this heterogeneity.
Let us examine the case of a tropical rain forest: for example, a ‘pristine’ Amazonian forest (Fig. 1). A great variety of species – from 150 to 250 different species of trees per hectare – can be observed almost everywhere. Usually, the area surrounding a particular tree is constituted of trees belonging to different species and their spatial distribution is more or less stochastic. This stochasticity is ensured by the fact that pollens (fertilization) and seeds (dispersion) are often transported by wind, water, or animals. These processes have mainly stochastic characteristics. Obviously, the resulting spatial distribution of the trees of a given species is generally not an ideally uniform one. Indeed, the mechanisms involved are not as perfect as a well-oiled roulette in a game of chance. Moreover, in some places, some groups of organisms are more adapted than other ones to edaphic conditions or local microclimates, so that their relative abundance may be higher than in other places. Nonetheless, spatial structure is the most often diffuse and species are mixed.
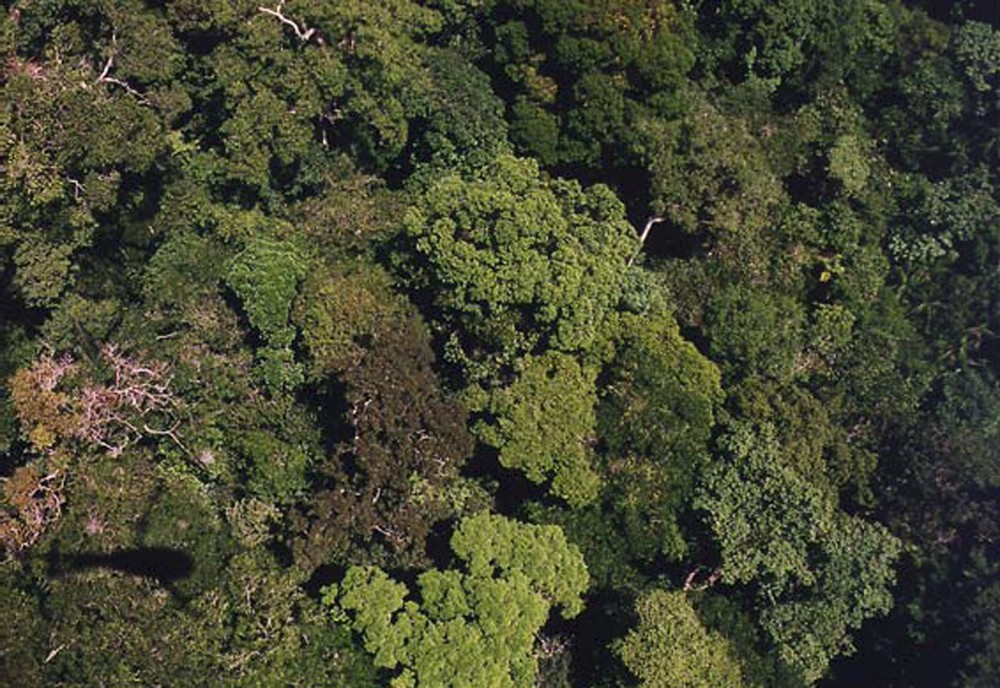
Aerial view of a tropical rainforest (southern French Guiana): on this scale, the level of biodiversity appears high. The shapes and colours of the trees' canopies are very different and correspond to several species. A quasi-random process might be responsible for the spatial distribution of species. It puts Hubbell's theory of biodiversity and biogeography on the similarity of the demographic properties of species in a community into perspective by introducing the idea of a quasi-random spatial distribution. This distribution is generated by ecological roulettes that mainly contribute to maintaining such diversity; otherwise, competition would lead to a decrease in the level of biodiversity. Biological and ecological roulettes may be the most important factors at the origin of the diversity. This kind of explanation also seems to be more accurate than the ‘niche theory’ – stating that diversity is the result of the heterogeneity of a milieu – but it does not exclude the importance of the concept of niche over a broader scale. (Photo: A. Pavé.) (For interpretation of the references to colour in this figure, the reader is referred to the web version of this article.)
How do such seemingly random distributions continue to exist in forests, which are among the oldest natural ecosystems? If we accept the conclusions drawn from classical theoretical ecology, the most competitive species, at least on a local scale, will win out in the long term, and we should be left with monospecific forests or, at least, large patches of homogeneous groups of trees. This is not the case. One explanation might be to suppose that the milieus (e.g., climate, soils) are highly heterogeneous. If this is true on a large scale, it is not true on a small scale; that is to say, for small groups of individuals.
To explain how biodiversity continues to exist over the course of many generations in one community, and then in an ecosystem, Hubbell [16] proposed the ‘neutral theory of biodiversity’. Volkov et al. [17] provide a brief summary of the main hypothesis: “Neutral theory in ecology builds on island biogeography, which asserts that an island or a local community approaches steady-state species richness where there is an equilibrium between the immigration of species from a much larger metacommunity source and the local extinction of species. The dynamics of local population are governed by birth, death and immigration events in both neutral and non-neutral models. Under neutrality, at large spatial and temporal scales, Fisher's log-series distribution is the expected steady-state distribution of relative species abundance at the speciation–extinction equilibrium in the metacommunity when per capita birth and death rates are density independent and the same for all species, and speciation is introduced.” In fact, the hypothesis of the constancy of demographic parameters between species is a cornerstone of this theory. This hypothesis is discussed at length, for example, in Chave [18] and Chave et al. [19]. This is also the case for the hypothesis of density independence.
Finally, neutral theory does not truly take into consideration the spatial distribution of individuals and its extraordinary heterogeneity on a small scale. That can be considered by introducing other mechanisms, already mentioned, such as the transportation of seeds by animals. Such a mechanism ensures the stochasticity of spatial distribution and enables biodiversity to continue to exist on this scale, permitting populations and species in the corresponding area to survive (Fig. 2). We must also consider entire ecosystems. Species have not evolved independently and co-evolution is not limited to two or only a few species. Co-evolution has to be considered as a global process that provides a balance between the ecological relationships that allow biodiversity to continue to exist. It is like a team, where individuals train together to win the game of life. In a nested ecosystem, individuals, populations belonging to particular species, and communities representing a set of species play the same game in a variable environment in the hope of winning together.
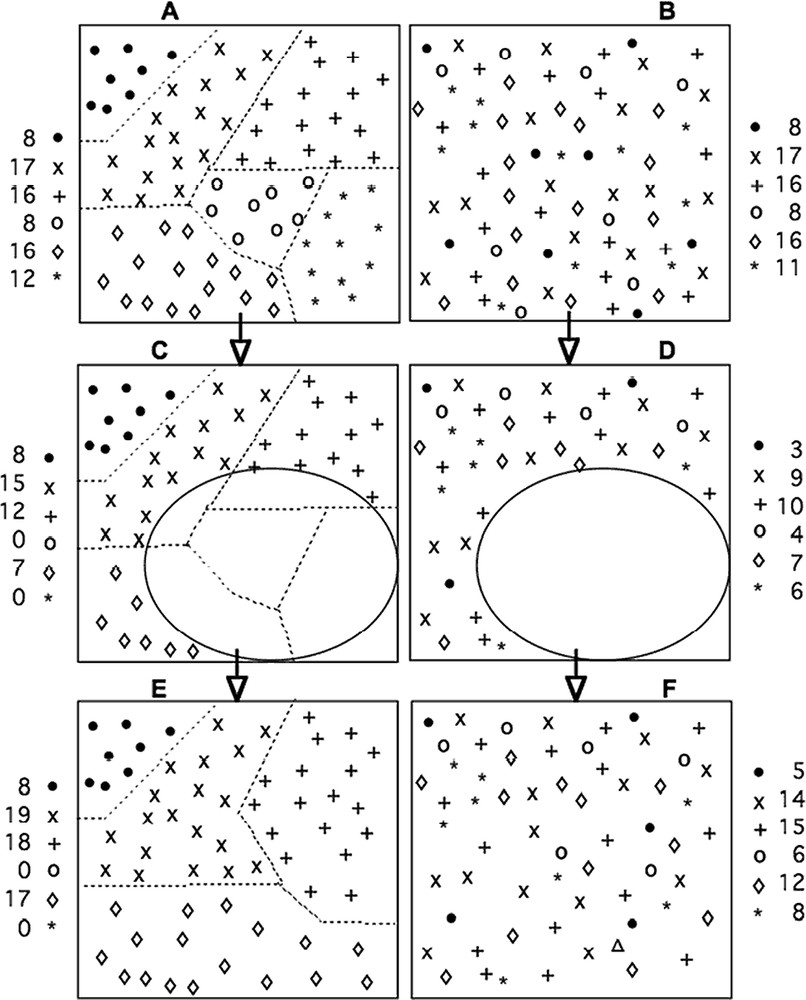
A theoretical example of the spatial distribution of trees in a diversified forest. Note that this didactic, idealized distribution has a practical basis; for instance, in [2], we developed this example by using ‘real’ data published in Dessart et al. [30], having a similar distribution. The points represent the individuals of different species (each symbol represents one of the six species). Plots A and B have the same diversity and relative abundance: the same number of species and same statistical distribution. However, in A, the spatial distribution is patchy and in B, it is random (like in most tropical rainforests). If we assume that a drastic event took place that killed individuals in one location (C and D), we immediately understand that the distribution A may lead to the disappearance of species (impact C). Conversely, in case D, there are survivors from all species. If we assume, for the purposes of simplicity, that there is no immigration (isolated area), and that the devastated area is only colonized by seeds of surviving plants, this leads to E (block distribution) and F (random distribution). E is less diverse then F. The stochastic distribution of individuals plays the role of a ‘life insurance policy’ for species. This scheme works on a local scale and is not in contradiction with Hubbell's neutral theory. However, it is well known that, if we consider such a plot in other climatic and/or edaphic conditions, the species set would be different from that proposed by the niche theory, where different species share the same niche (and thus co-exist or even cooperate). We propose this kind of mechanism to explain, at least in part, diversity peaks during evolution [26]. As such, this scheme contradicts neither the niche theory nor the neutral theory (which looks more like a null hypothesis than a realistic set of mechanisms). However, the introduction of the role of biological and ecological roulettes provides a complementary framework, within which spatial heterogeneity and biodiversity dynamics may be explained.
As a result, we must try to consider inner stochasticity from a positive point of view and, moreover, speak of the necessity of chance. The diversity that is produced by this stochasticity at all levels (i.e., organisms, populations and species, ecosystems) of the hierarchical organization of the living world is essential in that it provides living systems with the possibility of surviving environmental hazards or allows them to better exploit the resources in their milieu (e.g., the photosynthetic properties acquired by some species during evolution through endosymbiosis2).
Like Monte-Carlo methods and genetic algorithms developed by computer scientists to solve complex problems, the mechanisms that produce stochastic processes were selected during evolution. These mechanisms ‘insure’ truly ‘sustainable development’ for living things in an uncertain and unpredictable environment; moreover, such mechanisms enable those organisms that are well adapted to a particular environment to be selected. Selection also means that these organisms will be able to respond to environmental changes in a constant and optimum manner, and, in that way, these mechanisms work much like an algorithm, the goal of which is to find an optimum from a complex and multidimensional response, which is a function of environmental parameters. As such, the organisms are more resistant to large variations in environmental parameters (i.e., the response function has an optimum in a flat region of this function). Simultaneously, biological systems become more complex. On the one hand, this can be seen as being a consequence of the general flow of evolution; on the other hand, complexity, at least at a certain level, seems to provide an edge in terms of resilience (such as the complexity of the spatial distribution of trees in pristine, tropical forests). This complexity may also lead to emerging properties in the resulting systems (cf. footnote 4).
Eventually similarities between the biological structures and processes observed in different life systems, particularly organs and organisms, correspond to well-known convergence phenomena. This is the case, for instance, for the leaves of many plants: they have a similar geometry. In other words, the solutions to problems, such as the leaf form that best captures light, are very close. Thus, like Monte-Carlo algorithms, the random search for the optimal form, made possible by biological roulettes, leads to these similar geometries.
In the end, what is the nature of this game and what is its goal? In other words, how do living systems benefit from being subjected to such a large diversity of mechanisms generated or driven by chance? It is precisely, as François Jacob noted, to provide insurance for the long-term existence of organisms, populations, species, ecosystems, and, possibly, the biosphere itself. Diversity enables organisms to respond to stochastic environmental hazards. These responses, though they might look stochastic themselves, are largely the result of the inner mechanisms that produce diversity. It then becomes reasonable to assume that these mechanisms appeared spontaneously, and, because the main advantage they insure is the one of subsistence, that they were selected during evolution. It is well known that major and minor environmental hazards occurred (cf. next section) throughout this long history, which led, for example, to the disappearance of many species. Nevertheless, thanks to biological diversity, many others survived these disturbances that, over time, have been major factors in natural selection.
4 Biodiversity and the history of life: when did biological roulettes first appear?
When examining data gathered on past changes in biodiversity over a geological time scale, it is surprising to note the relative time duration between evolutionary stages. The time elapsed between the birth of the Earth and the emergence of life on our planet is relatively short (less than 700 million years, after the beginning – 4.5 billion of years ago). Then for a long time, the only living organisms were prokaryotes, or unicellular organisms. Eukaryotes, also mono-cellular, appeared at least 2 billion years after prokaryotes. Then it took a little more than 1 billion years before metazoans emerged (Fig. 3). Finally, during the Phanerozoic period (more or less during the last 600 million years), quantitative changes and variations in biodiversity appear to accelerate and are greater than the previous ones.
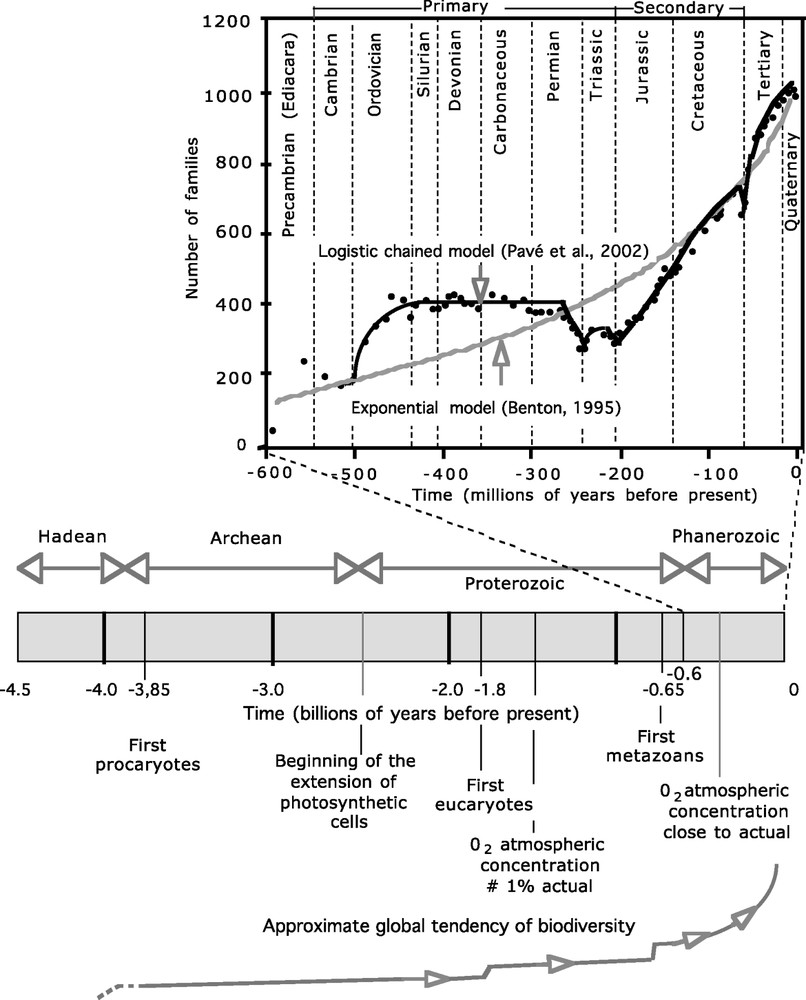
Since life began on Earth, biodiversity and bio-complexity have generally tended to increase (the dates are based on the first known fossils; obviously similar types of organisms had already appeared). Thus, for a long time, only single-celled organisms were present on Earth and only in the sea. The dearth of information available to us makes it difficult to estimate correctly biodiversity; if we base ourselves on a recent analysis of a stromatolitic reef [31]; it was already relatively high just after life began on Earth. However, it was relatively low if we compare this with evidence from the Phanerozoic Period, where the average increase is exponential (cf. Benton, 1995 [32], based on data from Sepkosky's famous database on marine fossils [24]); despite major anomalies, it can also be used as a model. We can then approximate the tendencies of biodiversity and biocomplexity all throughout geological time (lower curve). It is reasonable to assume that ‘breaking points’ or ‘periods’ of important diversification correspond to the emergence of new diversification mechanisms, particularly new ‘biological roulettes’.
Clearly, the appearance of new categories of organisms corresponds to qualitative breaks in the chain of evolutionary events that might result either from random events, taking place in the environment, or from the appearance of new and selected biological roulettes. If we assume that environmental perturbations were more or less uniformly distributed throughout history (by chance, even major ones are not so frequent) and possibly greater at the beginning, it would be reasonable to assume that major changes in living systems are related to the appearance of new mechanisms of diversification. It is, however, difficult to prove this by examining the geological record. The best arguments, then, are not those based on palaeontological data. By looking, as we did, at a detailed list of known living processes resembling random events, one could argue that these processes are possibly produced by complex biochemical, biological or ecological mechanisms.3 We should also mention that a great deal of attention has been paid to the great ‘extinctions’, and that their causes have been the subject of a major debate. Mainly, we should underline the extraordinary biological diversifications that followed all of these ‘catastrophic events’, as was the case, for example, for the five major extinctions and their aftermaths at the end of the Ordovician, Devonian, Permian, Triassic and Jurassic Periods.
Nonetheless, if it is not possible today to date the emergence of diversification processes from palaeontological data, it is possible to sketch out an organised sequence of events. One may assume that simple mechanisms, such as punctual mutations caused by environmental factors, appeared first. Then simple biological roulettes would have appeared, causing small pieces of DNA to be deleted or inserted. These mechanisms would have governed the transfer of sequences between organisms, and, eventually, made transpositions within the genome possible. It is reasonable to assume that elementary molecular mechanisms preceded sexual processes of reproduction and a fortiori corresponding behaviours. Finally, these events related to ecological interactions, such as pollination or seed dispersal, between organisms of different species could only come after the birth of those species and the formation of the ecosystems themselves. This sequential list provides at least one possible time-line along which biological roulettes may have emerged. There are various epigenetic processes and it is still too early to talk about when they first appeared during the history of life; however, if we compare DNA methylation and the role of RNA interference, for instance, it is reasonable to assume that the former is simpler than the latter and might have appeared first.
If diversification processes naturally lead to biodiversity over the long term4, biodiversity is also the consequence of the capacity of new living entities to find an environment that will allow them to survive and develop. Variants can subsist in organisms that are adapted to their biophysical environment. For this reason, we developed a global model, based on a logistical model similar to the one developed by Courtillot and Gaudemer [25], but which integrates a variable representing ecological niches and which includes increasing and decreasing components of the global dynamics of biodiversity [26]. It is also for this reason that we tried to understand better the mechanisms behind diversification and were led to assume that they are not only the consequence of environmental hazards. Therefore, we now assume that they are also produced by biological mechanisms, called biological roulettes. They emerged spontaneously, selected because they provide the simple advantage of enabling living systems – and even, throughout the history of the Earth, life itself – to continue.
5 Conclusion
In addition to examining the mechanisms that generate the smoothly deterministic functioning of the dynamics and structures of life systems (often seen as selected by necessity), it would be appropriate to identify the stochastic processes resulting from ‘natural roulettes’. They are mainly the biological and ecological processes working within living systems. They constitute the basis for most diversification processes and the means by which spontaneous biodiversity continues to subsist. We verified the plausibility of such a hypothesis by showing that, like a roulette wheel, a simple deterministic model of population dynamics could generate stochastic-like events [1]. They are essential to the emergence and permanence of biodiversity. Their efficiency means that they were probably selected during evolution. Furthermore, we can underline the fact that biological complexity is also a product of such processes.
Nevertheless, we must still identify and analyse them precisely. This will allow us not only to understand more about the way living systems function, adapt and evolve, but will also enable us to use some of them (e.g., life systems management and engineering, as discussed in [2] and [27]) for practical purposes. We have seen that diversity enables ecosystems to withstand environmental hazards; therefore, if we consider the case of forest systems' management, it would perhaps be of interest to plant trees belonging to diverse species, or simply to leave everything to ‘natural regeneration’. We could assist in this ‘natural regeneration’ by introducing seed disseminators into these forest systems to ensure the stochasticity of the spatial distribution of individuals as shown in Fig. 2 (right column). The overall resilience of the system would then increase. Another challenge is to limit diversification by either accelerating or slowing down the ‘spin’ of biological roulettes; for example, limiting microbial (e.g., viral) variability might make the immune system's response more efficient and thus aid in fighting infectious diseases. It is well known that chance is the major engine driving evolution. If, however, chance is produced at least in part by selected biological mechanisms, this adds another argument in support of this widely accepted theory, by introducing an explanation as to the origin of chance. Such a mechanism could also engender another similar mechanism that could also produce chance much like a self-catalytic process or a self-referenced algorithm. A new mechanism is selected if it presents a clear advantage; for instance, if it facilitates efficient diversification and, thus, the survival of living systems. In this case, older mechanisms might disappear if rendered useless or even harmful. It also contributes to amplifying diversification itself, and, in this way, providing greater ‘protection’, the way an insurance policy does, against those things that might damage living systems ‘for life’, as has been observed throughout the history of life on Earth since at least the Cambrian Period. On a smaller scale, a new mechanism preserves the natural continuance of such diversified systems as tropical rainforests. This might explain why biodiversity continues to exist in these ecosystems, whereas classical models of theoretical ecology assume that competition is an ecological mechanism leading to simplification. Briefly, biological roulettes and the chance that they generate are at the same time products and engines of evolution.
Based on these remarks, a general scenario can be proposed that is one drawn over the largest scales of space and time (i.e., the biosphere and evolution), but could equally be drawn for one of the smallest: an ecosystem.
- – The presence of life on the Earth is undeniable!
- – That many species are able to live together is the direct consequence of the fact that living systems have globally resisted major variations in the environment.
- – This global resistance has been made possible because there has been enough biodiversity throughout this history, making possible the survival of organisms more or less already adapted to a large range of environmental conditions.
- – Biodiversity results from processes that are the consequence of random environmental events, or, perhaps even more so, from biological and ecological roulettes.
- – Complexity is a consequence of diversification,5 but it also increases the global resilience of living systems and is then naturally selected or even sustained. It also endows these systems with newly emerging properties.
- – Diversification also allows living systems to colonize a large range of milieus; the resulting spread of species contributes to the global resilience of the biosphere.
All of this might explain the presence of life on Earth, and perhaps its absence on Mars: if life existed there in the past, which is a reasonable hypothesis, environmental conditions might have strongly changed before enough diversification processes were able to appear. Life could then not be maintained.
As evoked above, it is also possible that those diversification processes caused by biological roulettes have themselves progressively appeared and been diversified. The main argument supporting this idea is the tendency of general, non-linear diversity to increase, almost exponentially, since life emerged on this planet and, in particular, since the ‘explosions’ during the Ediacarian and Cambrian Periods (Fig. 3). It also provides a clear picture of the limits of our knowledge about the diversity of biological systems and of Man's ability to take action to manage it: a great many of the spontaneous processes leading to diversification resulting from natural evolution still remain beyond our ability to perceive them.
Acknowledgements
I would like to thank Michel Thellier and Jean-Claude Mounolou for their comments and discussions about this paper all throughout its writing. Many thanks also to Andrea Dejean for proofreading the English version.
1 We have chosen the generic expression ‘biological roulettes’ because it is illustrative of sophisticated mechanisms as we can imagine them, whereas other analogies based on games of chance, such as game of dice, seem to be too simple to represent these mechanisms.
2 Nowadays, it becomes admitted that the classical systematic classification of the living world is to be reconsidered. From genomic data, it appears three principal groups: Archæ, Bacteria, and Eucarya. By considering the history of cellular substructure, such as chloroplasts, it seems that they come from cyanobacteria incorporated by endosymbiosis mechanisms in heterotrophic cells. That could be the origin of plants, at least for the main part of them [20]. Then the phylogenetic organization of life might be not arborescent, but rather bushing out.
3 Today, the best example remains the periodic and chaotic oscillation of a compound produced in the glycolytic pathway (phosphofructuokinase). In 1982, Decroly and Goldbeter proposed a model for the corresponding reaction, which involves three variables. This model can exhibit oscillating solutions, which was expected, but also chaotic ones [21]. Experimental proof was obtained in 1997 by Nielsen et al. [22]. This story is well reported in [23].
4 We have to bear in mind that the estimation of biodiversity, over a geological time scale, depends on the quality of natural records and particularly of the fossilisation capabilities of organisms in a particular environment; this is why the most commonly used data are those collected and treated by Sepkosky on marine fossils [op. cit.].
5 Obviously, complexity increases with diversity (increase in the number of entities and relationships, and, in the case of living systems, in generally non-linear relationships).