1 Introduction
G protein-coupled receptors (GPCRs) mediate senses such as odour, taste, vision and pain [1] in mammals. In addition, important cell recognition and communication processes often involve GPCRs. Nearly 30% of total drug sales and prescriptions are directed at GPCRs [2], making them the most highly desired drug discovery targets by the pharmaceutical industry [3–5]. Among the GPCRs, dopamine receptors are ideal targets for treating schizophrenia and Parkinson's diseases. Dopamine receptors are members of structurally related G protein coupled receptors that share a high degree of homology to bovine rhodopsin [6]. However, a more recent report on human beta2 adrenergic G-protein-coupled receptor shows a highest degree of homology to D1 dopamine receptor [7] than the bovine rhodopsin. Five dopamine receptors have been identified and classified as D1-like (D1 and D5) and D2-like (D2, D3, and D4) based on their similarities to pharmacologically defined sites identified in brain tissue [8–10]. The D1 dopamine receptors are highly expressed in the nigrostriatal pathway where they play a key role in motor control [11]. Since the D1 dopamine receptor is more widespread than the other dopamine receptors and tends to show up in greater density, the development of improved remedies would be significantly enhanced with the availability of the 3D structure for the D1 dopamine receptor.
Scorpion venoms and their toxins have been extensively used as tools for understanding a wide range of pharmacological effects and the molecular basis of electrical excitability and neurotransmission [12,13]. They are rich sources of fascinating neuropeptides, which bind with high affinity and specificity to various ion channels. Notably, the Chinese scorpion Buthus martensi Karsch, which is a species belonging to the Buthidae family, has been used as a Chinese traditional medicine and is still used as a drug to treat neurological symptoms such as incomplete paralysis and mimetic paralysis [14]. A recent report state that scorpion neurotoxins target a wide variety of membrane-bound protein channels and receptors and that they are unique source of structural templates from which new therapeutic agents might be developed [15].
We report here the 3D structure of the long isoform of the human D1 dopamine receptor, predicted from the primary sequence. Following this, we have analyzed the interaction mechanism between the D1 dopamine receptor and the selected scorpion neurotoxins using docking simulations. To date, no data have been reported for a structural analysis of complexes between the D1 dopamine receptor and scorpion neurotoxins. In the present study, we modeled the D1 dopamine receptor using the human beta2 adrenergic G-protein-coupled receptor structure as a template that exhibits 37% sequence identity with the D1 dopamine receptor. Molecular docking simulations were performed between scorpion neurotoxins and the modeled D1 dopamine receptor. The role of conserved residues, binding affinity between the D1 dopamine receptor and scorpion neurotoxins, solvent accessibility changes, role of stabilization centres and stabilizing residues on docking simulations were computed.
2 Materials and methods
2.1 Data set
Atomic coordinates and crystallized structures of ten selected scorpion neurotoxins, 1AHO, 1B7D, 1DJT, 1DQ7, 1PTX, 1SN4, 1ZYW, 2A7T, 2I61, and 2SN3 [16–25] were obtained from the PDB at the Brookhaven National Laboratory [26]. All the structures were solved with resolutions, and the sequence identity among the majority of proteins in the dataset was less than 40%. Also these neurotoxins were selected so as all of them had 60–65 residues.
2.2 Molecular modeling of D1 dopamine receptor
We obtained the sequence version of the human D1 dopamine receptor from Swiss-Prot (http://expasy.org/sprot). Then a BLAST (http://www.ebi.ac.uk/blast2/index.html) sequence analysis was performed against the whole PDB, to select the correct template that could be used to generate a model of the D1 dopamine receptor. From the selected template, the three-dimensional (3D) structural model for the D1 dopamine receptor was generated by the biopolymer homology modeling software of Swiss-model/Deep view 3.7. The generated D1 dopamine receptor model was then checked with known agonists and antagonists.
2.3 Computation of conservation of amino acid residues
We computed the conservation of amino acid residues in each scorpion neurotoxins using the Clustal-W multiple alignment program [27].
2.4 Protein–protein docking
CHARMM force field [28] (license No. 070815-29) was used to minimize the structures of scorpion neurotoxins and of the D1 dopamine receptor before docking. Molecular docking was performed using protein–protein docking software GRAMM v. 1.03 [29]. The GRAMM v. 1.03 is the best protein–protein docking software certified in the CAPRI (Critical Assessment of PRediction of Interactions) test and the full results of CAPRI are made available on its website (http://capri.ebi.ac.uk). The algorithm used by GRAMM v. 1.03 performs Fast Fourier transformation methodology by employing smoothed potentials, refinement stage and knowledge-based scoring. We used a fine-grid projection of a softened Lennard–Jones potential function [30] and then the top 4000 grid-based predictions are subjected to a conjugate minimization in continuous 6D rigid body space with the same soft potential. One representative prediction for each minimum is stored and the number of initial predictions falling into this minimum is marked as the volume of the minimum. The average radius of such minima on our smoothed landscape is 5 Å.
2.5 Computation of individual residue–residue and overall binding free energies of the toxin–receptor complex
Interaction free energies are crucial for analyzing binding propensities in proteins. We computed binding affinity, desolvation free energy, electrostatic and contact free energies of docked structures using the program FastContact [31]. FastContact provides a fast estimate of the interaction free energy between two proteins. The interaction between two proteins is estimated as , where:
2.6 Computation of solvent accessibility changes in binding amino acid residues
Solvent accessibility is the ratio between the solvent accessible surface area of a residue in three-dimensional structure and that in an extended tripeptide conformation. We have analyzed the solvent accessibility of binding amino acid residues in the toxins as well as in the D1 dopamine receptor before and after the docking simulations. A majority of binding residues showed a change from an exposed state before binding to a buried state after binding. We obtained the solvent accessibility information using NetASA program [34].
2.7 Computation of stabilization center
Stabilization center residues are defined by considering the contact map of a protein. Two residues are in contact if there is at least one pair of heavy atoms with a distance less than the sum of the van der Waals radii of the two atoms plus 1.0 Å. A contact is considered long range if it is between residues that are separated by at least 10 residues in the amino acid sequence. Two residues are stabilization center elements if they are involved in long-range contacts and if at least one supporting residue can be found in each of the flanking tetrapeptides of these residues, in such a way that at least seven out of the possible nine interactions are formed between the two triplets [35,36]. We used the server for identifying the stabilization centers at http://www.enzim.hu/scide.
2.8 Computation of stabilizing residues
Stabilizing residues play an important role in the stabilization of proteins. We have computed the stabilizing residues in all 10-scorpion neurotoxins and for the D1 dopamine receptor using parameters such as surrounding hydrophobicity, long-range order, high conservation score, and if it belongs to a stabilization center. We used the public server available at http://sride.enzim.hu for this purpose [37].
3 Results and discussions
3.1 Model protein
Molecular modeling of the D1 dopamine receptor was achieved on the basis of the crystal structure of the human beta2 adrenergic G-protein-coupled receptor [7] solved at 3.4 0 Å (PDB ID 2R4R). The sequence identity between the D1 dopamine receptor and the Beta2 adrenergic G-protein coupled receptor is 37%. Detailed information about the human beta2 adrenergic G-protein-coupled receptor and the D1 dopamine receptor is depicted in Table 1. BLAST output showed that the human beta2 adrenergic G-protein-coupled receptor exhibited an excellent E-value, , and a bit score of 232. The alignment between the D1 dopamine receptor and the human beta2 adrenergic G-protein coupled receptor is shown in Fig. 1. The three-dimensional (3D) structural model for the D1 dopamine receptor was generated based on the human beta2 adrenergic G-protein-coupled receptor template by using the biopolymer homology modeling software of Swiss-model/Deep view 3.7.
Detailed information about the human beta2 adrenergic G-protein coupled receptor and the D1 dopamine receptor
GPCR | No. of amino acids | Molecular weight (KDa) | Gene name | Source organism | Swiss-Prot accession number | PDB ID |
Human beta (2) adrenergic receptor | 365 | 4.65 | ADRB2 | Homo sapiens | P07550 | 2R4R |
D1 dopamine receptor | 446 | 49.2 | DRD1 | Homo sapiens | P21728 | – |
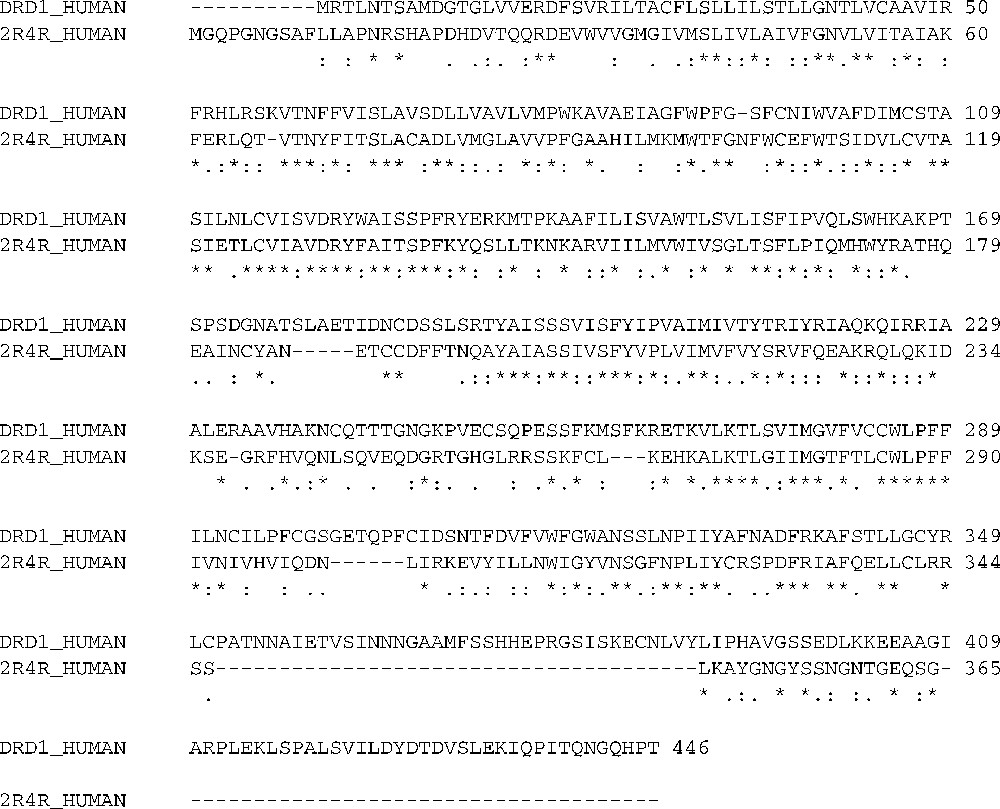
Sequence alignment result between the D1 dopamine receptor and the human beta2 adrenergic G-protein coupled receptor (2R4R).
The modeled D1 dopamine receptor structure was checked with known agonists and antagonists. The agonists are A77636, A68930, dihydrexidine (DHX), dinapsoline, dinoxyline and dopamine. The antagonists are LE300, SCH23390. We obtain the 2D structures of agonists and antagonists from Pubchem and the 2D structure was converted to 3D structures by using CORINA software. All of them were bound with modeled D1 dopamine receptor at their specific regions. We showed dopamine binds with the predicted structure of D1 dopamine receptor in Fig. 2. The geometric qualities of the models were assessed by PROCHECK. This provides a good validation of the predicted structure for the D1 dopamine receptor and of the predicted binding site of dopamine.
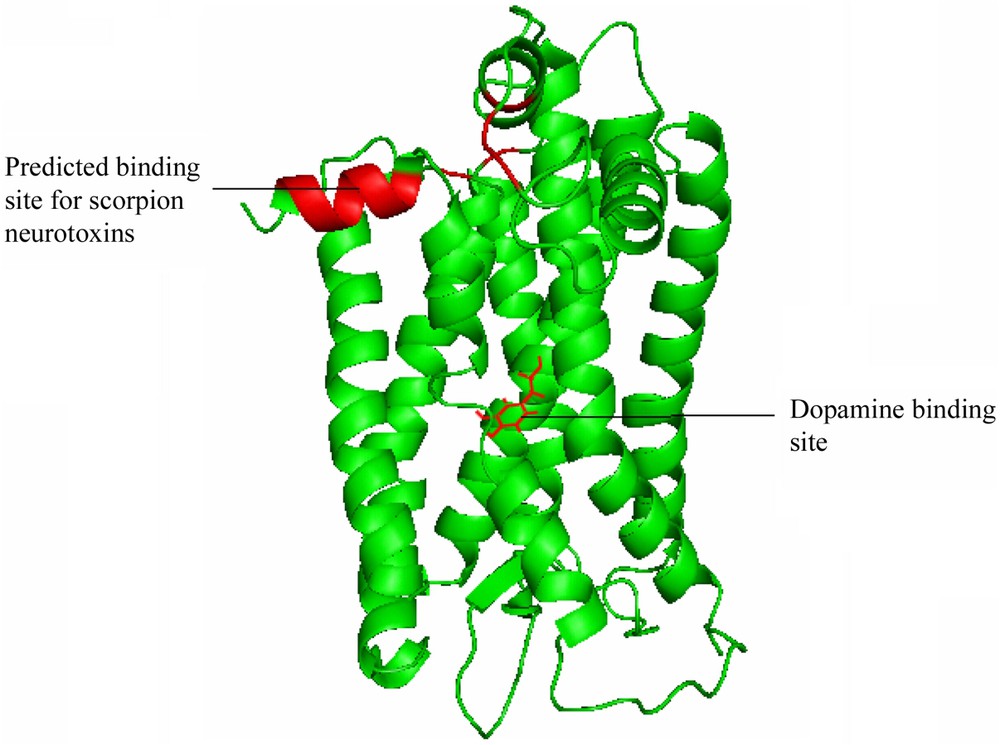
The predicted 3D structure of the human D1 dopamine receptor with 7 transmembrane helices; the predicted binding site of dopamine and the putative binding region for scorpion neurotoxins are shown in red colour. (For colours see the web version of this article.)
3.2 Conserved residues involved in the binding sites
We computed the conservation of residues using ClustalW multiple alignment program [27]. It was observed that highly conserved residues in scorpion neurotoxins are tyrosine, cysteine, glycine, leucine, phenylalanine and partially conserved residues are aspartic acid, asparagine, serine, lysine, and isoleucine. It is notable observation that these highly conserved residues were involved in the docking sites with the D1 dopamine receptor. Hence it can be thought that the conserved residues play a major role as docking sites for the toxin molecule. Among the major conserved residues in the toxin molecule, aspartic acid, lysine residues were involved in binding sites more frequently than others. The multiple alignment for all the scorpion neurotoxin is shown in Fig. 3.
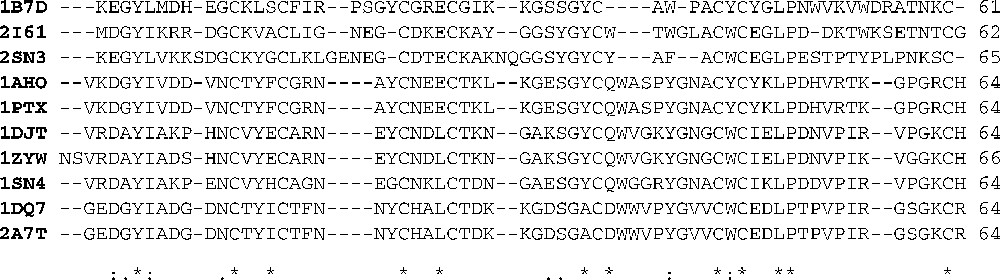
Multiple alignment of scorpion neurotoxins using clustalW.
3.3 Scorpion neurotoxins docked with D1 dopamine receptor
CHARMM force field [28] was used to minimize the structures of scorpion neurotoxins and of the D1 dopamine receptor before docking. All the ten scorpion neurotoxin selected for this study showed a good docking behaviour with the D1 dopamine receptor. The interface residues of the D1 dopamine receptor and the scorpion neurotoxins involved in binding are shown in Table 2. The docked complexes of the scorpion neurotoxin with the D1 dopamine receptor are shown in Fig. 4. It can be seen from Fig. 4 that there is a high degree of uniformity in the docking regions and also with the docking residues. We calculate the overall binding free energy for each of these ten complexes; the results are shown in Fig. 5. It was observed that the complex formed with neurotoxin (PDB ID 2A7T) shows the highest overall binding affinity. From the X-ray structure report of 2A7T neurotoxin [23], residues Glu(2), Asp(50), Arg(64) that protrude outwardly in this neurotoxin are the main residues for interaction with the receptor. This experimental result is well supported by our computational method for 2A7T. Also, this is the main reason for the highest binding affinity of this neurotoxin towards the D1 dopamine receptor. The complex formed with (PDB ID 2SN3) had the lowest binding affinity with the D1 dopamine receptor. Further, it could be seen from Fig. 5 that the binding free energies are almost similar for all other scorpion neurotoxins with the D1 dopamine receptor.
Interface residues of the D1 dopamine receptor and the scorpion neurotoxins involved in binding
Name | Interface residues involved in binding |
D1 dopamine receptor | Ile(10), Ala(22), Arg(26), Arg(28), Arg(31), Ser(32), Lys(33), Asn(36), Ser(41), Asp(79), Ser(83), Asp(96), Tyr(107), Arg(109), Lys(110), Thr(112), Lys(114), Val(122), Ala(123), Ser(127), Phe(236), Lys(241), Arg(242), Lys(245), Lys(248), Leu(250), Ser(251), Ile(253), Phe(313), Arg(314), Lys(315), Arg(314), Asp(96), Ser(239), Pro(304) |
1AHO | Lys(2), Asp(3), Tyr(5), Val(10), Lys(28), Lys(30), Cys(48), Tyr(49), Lys(50), Asp(53), Arg(56) |
1B7D | Lys(1), Glu(2), Lys(12), Arg(18), Gly(24), Asp(55), Lys(60), Cys(61) |
1DJT | Asp(3), Lys(8), Asn(11), Asp(24), Lys(28), Lys(32), Asp(53), Arg(58), Lys(62) |
1DQ7 | Glu(2), Asp(3), Asp(10), Lys(30), Asp(37), Thr(53), Lys(62) |
1PTX | Asp(8), Lys(28), Lys(30), Gly(31), Glu(32), Cys(36), Lys(50), Leu(51), Asp(53) |
1SN4 | Asp(3), Tyr(5), Ala(7), Lys(8), Glu(10), Glu(20), Asp(28), Gly(43) |
1ZYW | Asp(5), Tyr(7), Asp(10), Lys(30), Lys(34), Val(41), Lys(43) |
2A7T | Glu(2), Asp(8), Asp(10), Lys(29), Lys(30), Asp(32), Asp(50), Lys(62), Cys(63), Arg(64) |
2I61 | Lys(5), Arg(6), Asp(8), Lys(11), Asp(22), Lys(23), Lys(26), Gly(46) |
2SN3 | Lys(7), Leu(17), Glu(21), Asp(26) |
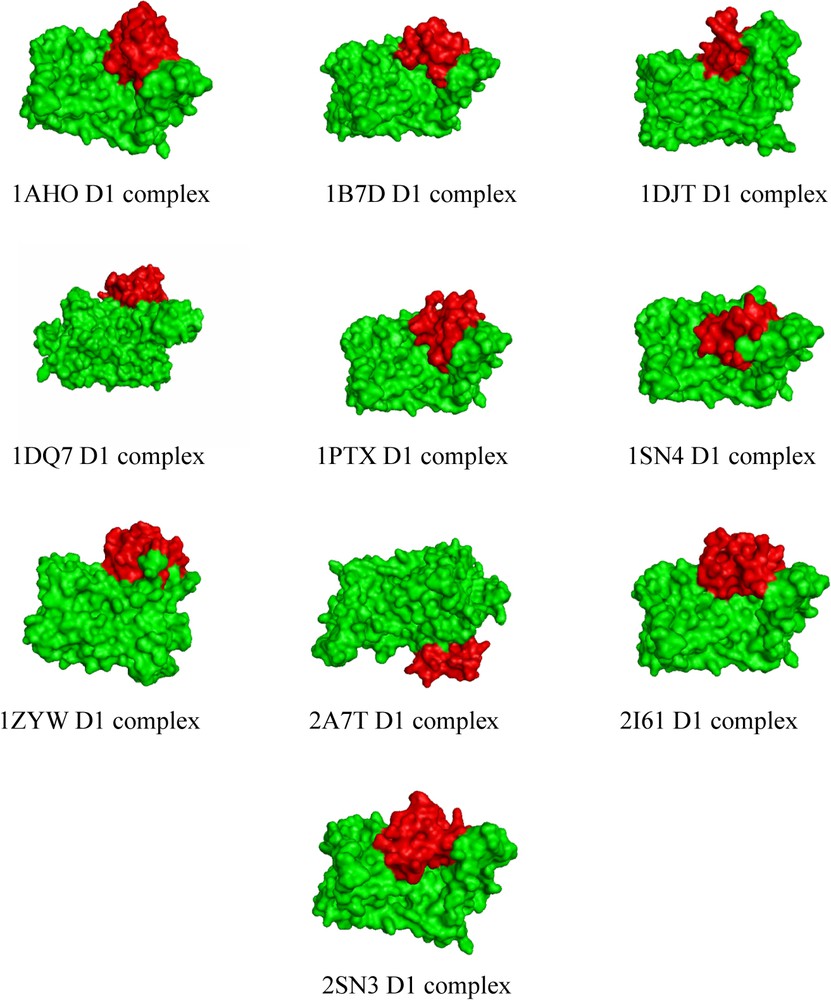
Docked complexes of the scorpion neurotoxin (red) with the D1 dopamine receptor (green). (For colours see the web version of this article.)
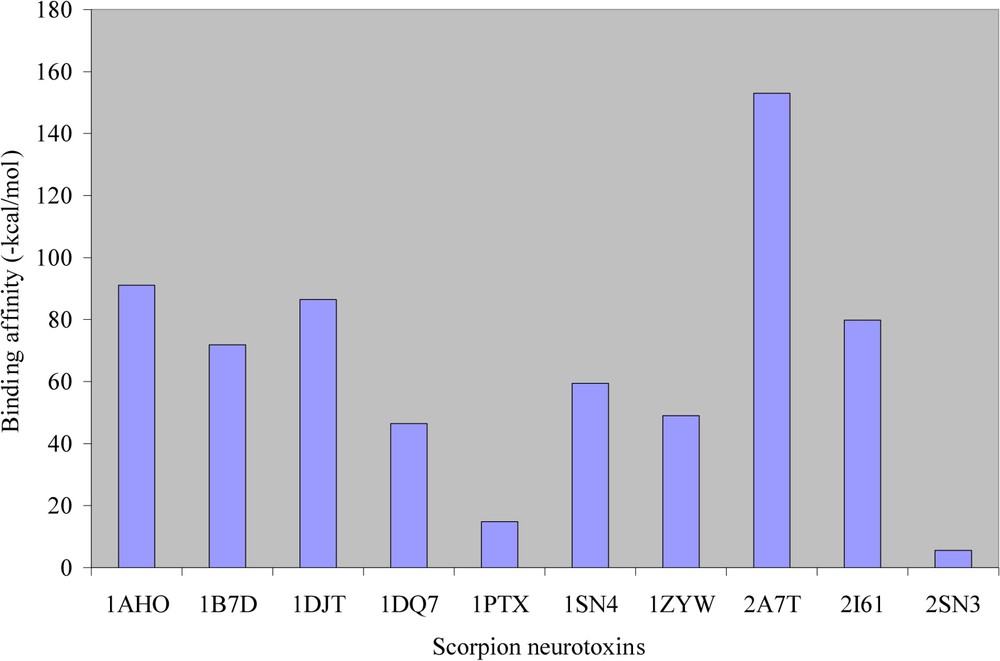
Overall binding affinities of scorpion neurotoxins with the modelled structure of the D1 dopamine receptor.
3.4 Residues with minimum and maximum binding free energies
Table 3 shows the minimum and maximum binding energies for individual residues after the formation of the complex. It can be seen from Table 3 that the minimum energy for binding ranges from 1.988 to 10.600-kcal/mol, while the maximum binding energy ranges from 0.481 to 1.903-kcal/mol. It can be observed that, except for 2SN3, all other binding energies are almost similar. Among the selected neurotoxins, 2A7T shows the highest binding affinity towards the D1 dopamine receptor. Our results on the binding effect of scorpion toxins with the D1 dopamine receptor and other GPCRs are correlated with the following experimental reports. Ismail et al. reported that scorpion Leiurus quinquestriatus venom blocked the dopaminergic receptors during envenomation [38]. Teixeira et al. reported a good experimental evidence for interaction between scorpion toxins with GPCR-like adrenergic and cholinergic receptors [39]. Scorpion venoms mediate their autonomic effects through direct agonist actions on post-junctional muscarinic M3 cholinoceptors and α-adrenoceptors that were reported by Matthew et al. [40]. Fernandes et al. proved the effects of scorpion toxin, tityustoxin on the release of [3H] dopamine of rat brain prefrontal cortical slices [41]. Scorpion toxins active in pancreatic secretion include those that act on ion channels (Na+, K+, and Ca2+), G Protein coupled receptors (GPCR), and signalling kinases related to protein kinase C [42].
Minimum and maximum binding free energy contacts between the D1 dopamine receptor and scorpion neurotoxins
PDB ID | Maximum binding free energy (-kcal/mol) | Minimum binding free energy (-kcal/mol) | ||||
Binding residues | Binding energy (-kcal/mol) | Binding residues | Binding energy (-kcal/mol) | |||
D1 dopamine receptor | Toxins | D1 Dopamine Receptor | Toxins | |||
1AHO | Ser(41) | Tyr(5) | 1.612 | Ser(251) | Arg(56) | 3.610 |
1B7D | Leu(250) | Tyr(43) | 1.460 | Lys(248) | Glu(2) | 5.927 |
1DJT | Ala(22) | Cys(46) | 1.313 | Lys(315) | Asp(53) | 9.038 |
1DQ7 | Ile(253) | Val(55) | 1.394 | Lys(315) | Asp(37) | 10.548 |
1PTX | Thr(112) | Lys(2) | 0.481 | Lys(114) | Asp(53) | 4.438 |
1SN4 | Arg(314) | Glu(10) | 1.215 | Lys(114) | Glu(20) | 14.821 |
1ZYW | Tyr(107) | Lys(43) | 1.052 | Lys(110) | Val(41) | 1.988 |
2A7T | Phe(236) | Cys(63) | 1.903 | Lys(241) | Asp(10) | 10.600 |
2I61 | Ser(83) | Asp(22) | 1.689 | Asp(79) | Lys(23) | 4.238 |
2SN3 | Arg(26) | Leu(17) | 0.048 | Arg(28) | Glu(21) | 2.029 |
3.5 Solvent accessibility and their desolvation energy
Solvent accessibility of all the residues in both toxins (Table 4) and the D1 dopamine receptor molecule (Table 5) before and after binding were computed with NetASA [34]. It can be seen from both tables that the solvent accessibility change is mainly from the exposed state before binding to the buried state after binding. Moreover, such changes are comparatively much more for toxin molecules than for the receptor molecule. We also computed the desolvation energies before and after the binding by using the formula:
Solvent accessibility and desolvation energy of scorpion toxins before and after complex formation
PDB ID | Binding residues | Solvent accessibility (Ligand) | Desolvation energy | |||
Receptor | Toxin | Before binding | After binding | Before binding | After binding | |
1B7D | Asp(96) | Lys(12) | Exposed | Buried | +8.519 | −3.695 |
1B7D | Ala(123) | Cys(61) | Exposed | Buried | NA | −2.636 |
1B7D | Val(122) | Cys(61) | Exposed | Buried | NA | −2.368 |
1DJT | Lys(315) | Asp(53) | Exposed | Buried | +5.291 | −9.038 |
1DJT | Arg(314) | Asp(53) | Exposed | Buried | +2.678 | −3.103 |
1DJT | Lys(245) | Asp(53) | Exposed | Buried | +3.582 | −2.556 |
1DJT | Phe(313) | Arg(58) | Exposed | Buried | −1.441 | −2.212 |
1DJT | Arg(314) | Asp(53) | Exposed | Buried | NA | −3.103 |
1PTX | Lys(33) | Glu(32) | Exposed | Buried | +3.479 | −1.638 |
1PTX | Ser(32) | Lys(50) | Exposed | Buried | +8.039 | −1.293 |
1PTX | Asn(36) | Glu(32) | Exposed | Buried | +3.478 | −0.696 |
1PTX | Asp(96) | Lys(50) | Exposed | Buried | +7.405 | −1.209 |
1PTX | Ser(239) | Cys(36) | Buried | Exposed | −0.647 | −1.426 |
1SN4 | Arg(109) | Asp(28) | Exposed | Buried | +3.239 | −1.672 |
1SN4 | Arg(28) | Gly(43) | Buried | Exposed | +3.447 | −1.385 |
2A7T | Arg(31) | Asp(50) | Exposed | Buried | +4.533 | −3.721 |
2I61 | Ser(127) | Gly(46) | Exposed | Buried | NA | −2.342 |
2I61 | Pro(304) | Arg(6) | Buried | Exposed | +5.814 | −3.231 |
2SN3 | Arg(28) | Glu(21) | Exposed | Buried | +1.970 | −4.029 |
2SN3 | Arg(31) | Glu(21) | Exposed | Buried | +0.903 | −0.888 |
2SN3 | Arg(26) | Glu(21) | Exposed | Buried | +0.873 | −0.072 |
Solvent accessibility and desolvation energy of receptor before and after complex formation
PDB ID | Binding residues | Solvent accessibility (Receptor) | Desolvation energy | |||
Receptor | Toxin | Before binding | After binding | Before binding | After binding | |
1DJT | Arg(242) | Asp(53) | Exposed | Buried | +3.721 | −2.131 |
2A7T | Ile(10) | Asp(10) | Buried | Exposed | +7.479 | −11.531 |
2A7T | Lys(241) | Asp(8) | Buried | Exposed | +7.410 | −5.740 |
3.6 Computation of stabilization center in binding residues
We computed the stabilization center for the complex using web server Scide [35]. The results of the stabilization center are shown in Table 6. One or more residues act as stabilization centre(s) that were also involved in the binding with the receptor shown in bold in Table 6. This can be seen in all the scorpion neurotoxins, except 1PTX and 2SN3. Among the selected neurotoxins, 2A7T has five residues, acts as stabilization center, and is also involved in binding with the D1 dopamine receptor. On the other hand, the neurotoxin 2SN3 has no residues that can act as stabilization center and are involved in binding. From this result, we concluded that stabilizing centers may enhance the stability of the complex and also may support the docking process.
Stabilizing centers involved in binding
PDB ID | Stabilizing centers in complex |
1AHO | Val(10), Thr(13), Tyr(49), Lys(50), His(54), Lys(2), Gly(4), Tyr(5), Ile(6), Tyr(14), Phe(15), Gly(34), Ala(45), Cys(48), Tyr(49), Lys(50), Leu(51), Pro(52), Asp(53), Val(55), Arg(56), Thr(57) |
1B7D | Gly(10), Leu(13), Ser(14), Cys(15), Phe(16), Arg(18), Gly(32), Pro(40), Ala(41), Gly(46), Asn(49), Trp(50), Trp(54) |
1DJT | Arg(2), Tyr(5), Ile(6), Ala(7), His(10), Asn(11), Val(13), Tyr(14), Glu(15), Cys(16), Ile(57), Arg(58), Lys(62), Cys(63) |
1DQ7 | Glu(2), Ile(6), Asp(10), Asn(11), Thr(13), Tyr(14), Ile(15), Cys(16), Glu(49), Asp(50) |
1PTX | Lys(2), Asp(3), Gly(4), Tyr(5), Ile(6), Val(10), Thr(13), Tyr(14), Phe(15), Cys(16) |
1SN4 | Arg(2), Tyr(5), Ile(6), Ala(7), Glu(10), Val(13), Ile(49), Lys(50), Asp(54), Val(55), Pro(56), Ile(57), Arg(58) |
1ZYW | Val(3), Arg(4), Tyr(7), Ile(8), Ala(9), Asp(10), Asn(13), Tyr(16), Glu(17), Arg(20), Asn(21) |
2A7T | Ile(6), Asp(10), Asn(11), Thr(13), Ile(15), Asn(19), Cys(22), Lys(30), Ala(35), Cys(36), Val(45), Glu(49), Asp(50), Leu(51), Pro(54), Val(55), Ile(57), Lys(62), Cys(63) |
2I61 | Gly(2), Tyr(3), Cys(10), Ala(13), Cys(14), Leu(15), Gly(17), Cys(21), Asp(22), Ser(31), Gly(33), Tyr(34), Trp(38), Gly(39), Leu(40), Ala(41) |
2SN3 | Asp(10), Thr(13), Val(49), Ala(50), Met(54) |
3.7 Computation of stabilizing residues in binding residues
We computed the stabilizing residues in the scorpion neurotoxin molecules and also in the complex using web server Sride [37]. The results of the stabilizing residues are shown in Table 7. Cys(48) in 1AHO, Leu(51) in 1PTX and Tyr(7) in 1ZYW were found as both stabilizing residues in the toxins and were also involved in binding. Ile(6) in 1DJT and 2A7T and Val(45) in 2A7T were found to be stabilizing residues in both toxins and also in the complex. This behaviour could not be generalized for other toxin molecules in the sense that certain residues that act as stabilizing residues in the toxin were not seen as the stabilizing residue when they formed the complex with the D1 dopamine receptor molecule. However, the complex formed could be considered to be quite stable as the results from the stabilizing residue were very favourable.
Stabilizing residues involved in binding
PDB ID | Stabilizing residues | Stabilizing residues in complex |
1AHO | Ala(45), Cys(48), Leu(51) | NA |
1B7D | Cys(15), Ala(41) | NA |
1DJT | Tyr(5), Ile(6), Gly(45), Cys(48) | Ile(6), Ala(7), Gly(45) |
1DQ7 | Ile(6), Val(45) | NA |
1PTX | Ala(45), Cys(48), Leu(51) | NA |
1SN4 | Ile(6), Ala(45), Leu(51) | NA |
1ZYW | Tyr(7), Ile(8), Ala(9), Cys(38), Gly(47), Leu(53) | NA |
2A7T | Ile(6), Cys(22), Val(45) | Ile(6), Cys(22), Cys(36), Val(45) |
2I61 | Gly(2), Tyr(3), Cys(21), Gly(33), Ala(41), Cys(44) | NA |
2SN3 | Cys(48) | NA |
4 Conclusions
From the results obtained in this work, the human beta2 adrenergic G-protein-coupled receptor is the best template for modeling D1 receptor as on date. We also report that the scorpion neurotoxins grossly appear to dock on the modeled structure of the D1 dopamine receptor. The overall binding affinity was found to be maximum for the toxin molecule with a PDB ID 2A7T and minimum for a molecule with a PDB ID 2SN3. A uniform docking was observed in all toxin-receptor complexes. Mainly, aspartic acid and lysine residues of the toxins were involved in the binding with the D1 dopamine receptor. The residues in the toxin molecules were mainly in the exposed state, but on complex formation with the D1 dopamine receptor, these residues went into the buried state from the exposed state. This was also accompanied by a sharp decrease in the desolvation energies, thus stabilizing the complex. Most of the residues in the toxin molecule were present as stabilization center before and after the complex formation, but, on the other hand, the residues that were stabilizing residues in the toxin molecule lost this property on complex formation. Thus it could be duly concluded that a stabilization center for the complex molecule may play a more important role than the individual stabilizing residues during the formation of the complex. This strategy may be helpful for structural characterization of macromolecular complexes when a high-resolution structure of a complex cannot be obtained by either NMR or X-ray crystallography experiments. Overall we emphasize that the toxin-D1 dopamine receptor complex structures obtained in this study are acceptable and can be used in guiding the design of future biological studies and the GPCR-based drug design targets.
Acknowledgements
The authors thank the Management of Vellore Institute of Technology University for providing the necessary facilities to carryout this work. We also thank all the reviewers for giving useful suggestions and comments on our manuscript.