1 Introduction
Potassium is the most abundant inorganic cation in the cytoplasm of all organisms. In plants, it plays a crucial role in osmoregulation, turgor maintenance and cell expansion [1]. Sodium ion can compete with K+ and inhibit transport and metabolic processes depending on K+. Since Na+ is frequently present at higher concentrations than K+ in soils, most plants must have transport systems with high selectivity for K+ against Na+ [2]. Plants also possess transport systems that compartmentalize Na+ into the vacuole. This is an essential function in protecting the cytosolic biochemical machinery and ensuring a balance against the low extracellular osmotic potential created by salt stress [3,4]. Plants feature both inter-specific and intra-specific variations for K+ and Na+ transport. NaCl-tolerant varieties generally maintain a high cytosolic K+/Na+ ratio, when they are in presence of salty medium [5–9]. Considering the K+ vs. Na+ selectivity as the ratio between the K+/Na+ ratio in leaves and the K+/Na+ ratio in the soil solution, a diversity analysis performed in A. thaliana evidenced a strong correlation between salt tolerance and K+ vs. Na+ selectivity [10]. The two accessions displaying extreme phenotypes were Columbia-0, which showed a 50% reduction in shoot dry weight and a K+ vs. Na+ selectivity of 1 in response to salt stress, and NOK2, which showed no reduction in shoot dry weight and a K+ vs. Na+ selectivity of 5.
The causes of the K+ vs. Na+ selectivity remain unclear. At least 35 genes present in the Arabidopsis genome are thought to encode various K+ channels or transporters [11]. The actual functions of some of these genes have been inferred from reverse genetic approaches, in which gene disruption was associated with lower K+ content and reduced cell or whole plant growth as compared to wild type [12–15]. Passive uptake of K+ into roots is mediated by K+-selective channels located on the plasma membrane of root cells such as AKT1 [12,14,16,17]. AKT1 disruption inhibits Arabidopsis growth at low K+ concentration [13]. This gene is differently regulated by salt in salt-tolerant and salt-sensitive rice varieties [8]. Potassium uptake is also ensured by the HAK5 gene. This transporter contributes to high-affinity potassium uptake in Arabidopsis roots [17]. Long distance transport of K+ involves SKOR [18] and AKT2 [19], two channels involved in K+ secretion into the xylem in roots and into the phloem in shoots, respectively. Both AKT1 and SKOR are present at loci that coincide with QTLs for K+ content in Arabidopsis leaves [20].
Information relative to the transporters involved in sodium transport is more limited. Vacuolar compartmentalization of Na+ is achieved by the action of Na+/H+ antiporters present on the tonoplast. AtNHX1 is one of these antiporters; it can mediate Na+ and K+ coupled transport in vacuoles [21] and its over-expression improves plant salt tolerance [21–23]. AtHKT1 has been shown to control Na+ homeostasis and to regulate potassium nutrient status in planta [24]. Recently, another Na+/H+ antiporter (NHX5) has been identified as playing a role in salt tolerance by controlling the accumulation of Na+ and K+ in shoots [25]. Sodium can also be moved out of the cell by SOS1, which encodes a plasma membrane Na+/H+ antiporter controlling long distance Na+ translocation from root to shoot in Arabidopsis. Over-expressing SOS1 in this species increases salt tolerance [26].
The purpose of this study was to explore the combined effects of a mild salt stress and increasing K+ supplies on the two Arabidopsis thaliana natural accessions, Columbia-0 and NOK2, at the phenotypic and molecular levels. A semi-quantitative RT-PCR method was used to monitor changes in the transcript abundance of the AKT1, AKT2, SKOR, NHX1 and SOS1 K+ and Na+ transporters in relation to the varying Na+ and K+ treatments.
2 Materials and methods
Seeds of NOK2 and Columbia-0 accessions provided by the Nottingham Arabidopsis Stock Centre (references N1403 and N907, respectively), were grown in pots containing a 1:2 (v:v) mixture of sand and peat, and placed in a culture chamber with a 12 h photoperiod (150 μmol m−2 s−1 PAR). The substrate contained 4.9 mg/Kg of K+ and 4.6 mg/Kg Na+. The seedlings were irrigated with distilled water during the first 10 days, and then with a nutrient solution [27] diluted to the quarter and composed of: 1.0 mM Mg SO4, 0.625 mM, KH2PO4, 0.5 mM Ca(NO3)2; 1.25 mM NH4NO3; 0.04 μM MnSO4, 0.5 μM ZnSO4, 0.05 H3BO3, 0.02 MoO3 et 3 μM FeEDTA, during 13 days. The first harvest was then performed. The plants were thereafter irrigated with the same nutrient solution modified to contain 0.1, 0.625 or 2.5 mM final potassium concentration and 0 or 50 mM NaCl. In the control medium, potassium was added as 0.625 mM KH2PO4. In the treatment with the lowest K+ concentration, potassium was added as 0.1 mM KH2PO4, and the medium was supplemented with 0.525 Na H2PO4 to maintain P level. Seventeen days later, plants were harvested. For RT-PCR analyses, samples were frozen in liquid nitrogen and stored at . Leaves and roots were analyzed separately.
2.1 Phenotypic and elemental analyses
Fresh and dry matter of leaves and roots were determined. For each rosette, all the leaves were cut and photographed, and the individual leaf surface areas were measured using the Optimas® software. Roots were collected by submerging the whole root system with the substrate attached to it in 120 ml distilled water. Three rinsing steps were sufficient to get rid of the substrate and rinse the roots. Roots and leaves of each plant were then separately digested in 0.1N HNO3. The concentrations of K+ and Na+ in the clear extracts were measured by flame photometry (Jenway PFP7, using butane air flame).
According to [28], rate of ion net uptake () was calculated for each time interval, as
2.2 Statistics
Data are presented as the mean of nine plants for each treatment. Significant differences between treatments were analysed using ANOVA and mean comparison with Duncan post hoc test (Statistica®). Values were calculated at the probability level.
2.3 RNA isolation
Plant material (3 plants pooled) was ground in liquid nitrogen, then resuspended in a 0.1 M Tris-HCl, pH 9 buffer containing 1% (w/v) SDS, 10 mM EDTA and 50 mM β-Mercaptoethanol. The mixture was extracted twice with phenol: chloroform:isoamyl alcohol (25:24:1, v:v:v). One tenth volume of 3M sodium acetate pH 5.2 and 2.5 volumes of ethanol were added to the supernatant and the mixture was left on ice for at least one hour for nucleic acid precipitation. After centrifugation at for 20 min, the pellet was resuspended in deionised water. For RNA precipitation, one volume of 4 mM LiCl was added and the mixture was placed on ice for a minimum of two hours. After 45 min of centrifugation, RNA pellets were air-dried for 15 to 30 min, and resuspended in diethyl pyrocarbonate-treated water. RNA was quantified by spectrophotometry. To ensure the quality of RNA, samples were assessed by formaldehyde-denaturing agarose gel electrophoresis.
2.4 Semi-quantitative RT-PCR
For reverse transcription, 1 μg RNA was incubated with 0.5 μg oligo-dT at 70 °C for 10 min. The reaction mixture was then brought to 50 mM Tris-HCl, pH 8.3, 75 mM KCl, 3 mM MgCl2, 10 mM DTT, 0.5 mM each dNTP and 200 U M-MLV reverse transcriptase (Promega) and incubated at 42 °C for 1 hour. The reaction was stopped by a 15 min long incubation at 70 °C. PCR reactions were conducted using an Eppendorf thermal cycler at 94 °C for 2 min to denature the samples, followed by 30 PCR cycles (30 s at 94 °C, 45 s at 60 °C, 1 min at 72 °C), and a 5 min final extension step at 72 °C. The number of amplification cycles (30) was determined to ensure that all amplification reactions were stopped in the exponential phase. The primers cited in Table 1 were designed for the gene-specific amplification of transcripts. They were designed to span an intron to distinguish PCR products generated from cDNA versus genomic DNA. The PCR reaction was resolved by electrophoresis on a 1 to 2% (w/v) agarose gel stained with ethidium bromide. Gel images were photographed and quantified using Image J version 1.37 software for Windows. The relative intensity of each band in each sample was quantified using spot densitometry and the data were calibrated against the quantities of the Ef-1α gene. mRNA accumulation measurements were done in triplicate for all the genes. mRNA accumulation measurements were done in triplicate for all the genes.
Forward and reverse specific primers used for RT PCR amplification and size of the amplified fragment.
Gene | Primer sequence () | Product length (pb) | ||
Genomic DNA | cDNA | |||
NHX1 At5g27150 | Forward | ACCGGTCTGATAAGTGCGTATGTTATC | 1464 | 911 |
Reverse | GTTACCCTCAAGCCTTACTAAGATC | |||
SOS1 At2g01980 | Forward | CAAATAAAATGACGACTGTAATCGAC | 1551 | 966 |
Reverse | GTCCTTGCAAATGCAGCATAAAAC | |||
AKT1 At2g26650 | Forward | CCGCAACTTCAACTACTTTTGGGTCCGATGCG | 591 | 510 |
Reverse | GGGAGTGCATCAAGAGTTTCTTGCTGTTGCAG | |||
AKT2 At4g22200 | Forward | CAGGAGAAATATAATGGACCTCAAG | 408 | 294 |
Reverse | GGGTAAACCCACGCAGAGTATG | |||
SKOR At3g02850 | Forward | CGTCGAGCTTTATTGCACTCAC | 510 | 431 |
Reverse | GCAATCCGCAAATGTCCGGTAATCTGACC | |||
Ef1α At1g07920 | Forward | ATGGGTAAAGAGAAGTTTCACATC | 830 | 730 |
Reverse | CCAATCTTGTAGACATCCTGAAG |
3 Results
3.1 Growth responses of the Columbia-0 and NOK2 A. thaliana accessions to the combination of variable potassium nutrition and NaCl treatments
The Columbia-0 and NOK2 accessions were not equally sensitive to increases in the K+ nutrition (Fig. 1). Whether NaCl was present or not in the culture medium, growth of Columbia-0 plants was not significantly altered in response to the increase in K+ concentration in the medium. In contrast, when the K+ concentration in the medium was increased from 0.1 mM to 2.5 mM, the final whole plant biomass of the NOK2 accession increased by ∼15% in cases where no NaCl was present and by ∼30% in the presence of 50 mM NaCl. The growth decrease observed for both accessions in response to salt at 0.1 mM K+ was suppressed upon increase in the K+ supply only in NOK2 (Fig. 1). A protective effect against NaCl stress caused by increasing the K+ supply was thus demonstrated only for the NOK2 accession.

Combined effect of increasing K+ concentration in the culture medium and applying or not a 50 mM NaCl treatment on growth of Columbia-0 (Col) and NOK2 plants. The 0.1, 0.625 and 2.5 mM K+ and 0 or 50 mM NaCl treatments were applied to 23 day-old individual plants and lasted 17 days. 50 mM NaCl: black symbols; 0 mM NaCl: white symbols. NOK2: triangles; Columbia-0: circles. Means ± confidence intervals (n = 9; p = 0.05).
Dissecting the growth response to understand what would be the cause of the observed differences between the two accessions did not give a clear answer. The total leaf surface per plant increased by (Fig. 1) in response to the increase in K+ supply for both accessions. The origin of this increase was different for the two accessions. Columbia-0 produced a greater number of leaves and showed symptoms of necrosis while NOK2 produced larger leaves without necrosis (Fig. 1). These responses were irrespective of the presence or absence of NaCl in the medium. A major difference between the two accessions was the leaf thickness. It was markedly higher for NOK2 than for Columbia-0, and increased by under NaCl stress in NOK2 while it decreased by in Columbia-0 (Fig. 2). In addition, increasing the K+ supply did not significantly change the leaf thickness in NOK2, while it resulted in a decrease in the leaf thickness in Columbia-0. The water content was also significantly differentially altered in an accession-dependent way, mainly in response to the presence of NaCl in the culture medium (Fig. 2). The NOK2 water content was lower than the Columbia-0 one when no NaCl was added to the culture medium. Upon NaCl stress, it reduced by only 20% while the Columbia-0 one reduced by more than 50%, so that the Columbia-0 water content was lower than the NOK2 one upon NaCl stress. In summary, the positive effect on growth of the increase in the K+ supply could only be observed for the NOK2 accession, more particularly when the plants were grown in presence of NaCl in the culture medium. The origin of this phenotype was probably that increasing the K+ supply resulted in an increase in the leaf surface area together with the preservation of leaf hydration and leaf thickness, which positively discriminated NOK2 in comparison to Columbia-0.
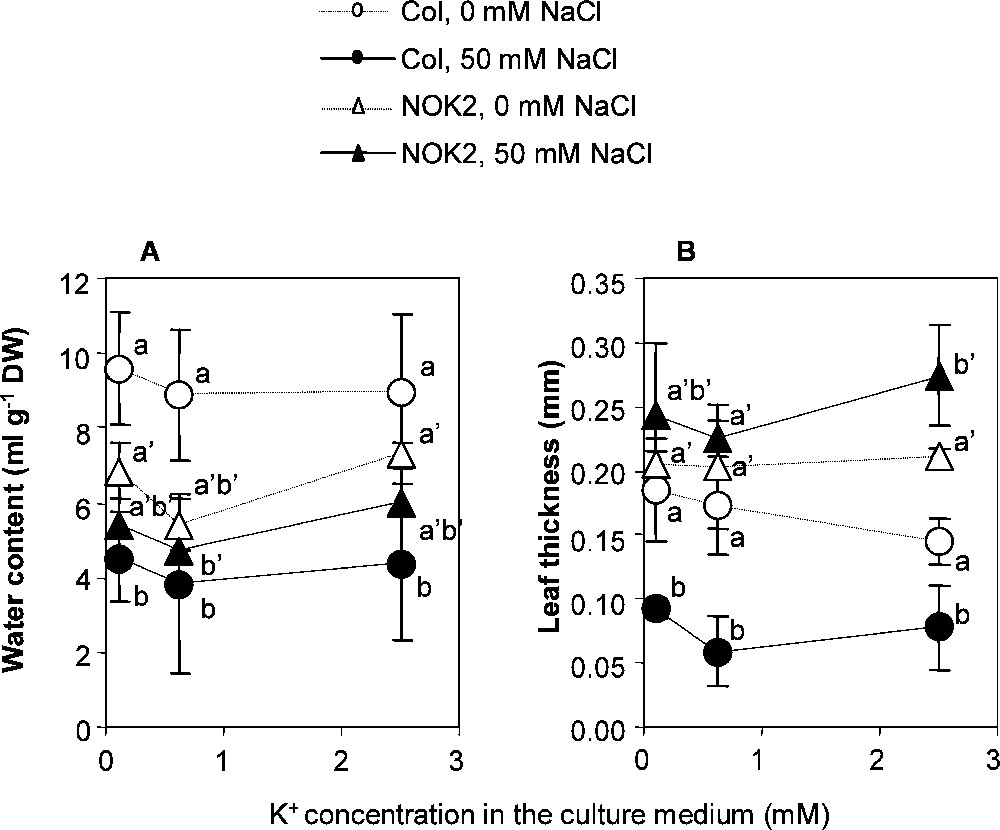
Combined effect of increasing K+ concentration in the culture medium and applying or not a 50 mM NaCl treatment on leaf water content and leaf thickness of Columbia-0 (Col) and NOK2 plants. The 0.1, 0.625 and 2.5 mM K+ and 0 or 50 mM NaCl treatments were applied to 23 day-old individual plants and lasted 17 days. 50 mM NaCl: black symbols; 0 mM NaCl: white symbols. NOK2: triangles; Columbia-0: circles. Means ± confidence intervals (n = 9; p = 0.05).
3.2 Effect of combining variable potassium nutrition and NaCl treatments on the K+ and Na+ net uptake and accumulation in Columbia-0 and NOK2
Leaf K+ content was strongly dependent on the level of the K+ supply to the culture medium (Fig. 3), increasing in most cases by to in response to an increase from 0.1 to 2.5 mM in the K+ concentration in the culture medium. The only exception was for Columbia-0 plants submitted to the-NaCl treatment: in this case, the leaf K+ content augmented by only 55% in response to medium enrichment in K+. The increase in leaf K+ content was correlated to a decrease in the leaf Na+ content in NOK2 plants submitted to the NaCl treatment, but it had no impact on the leaf Na+ content in Columbia-0 plants submitted to the same NaCl treatment (Fig. 3). Notwithstanding the variation in the level of the K+ supply, NOK2 plants accumulated ∼50% more K+ and twice less Na+ in their leaves than Columbia-0 plants, so that the K+/Na+ concentration ratio varied from 0.18 to 0.37 in NOK2, and from 0.07 to 0.11 in Columbia-0.

K+ and Na+ accumulation in Columbia-0 (Col) and NOK2 plants. The 0.1, 0.625 and 2.5 mM K+ and 0 or 50 mM NaCl treatments were applied to 23 day-old individual plants and lasted 17 days. A, C: leaf K+ and Na+ contents at the end of the treatments. D, E: net K+ and Na+ uptake during the 17-day long treatments. 50 mM NaCl: black symbols; 0 mM NaCl: white symbols. NOK2: triangles; Columbia-0: circles. Means ± confidence intervals (n = 9; p = 0.05). The small amounts of Na+ absorbed from solutions without added NaCl are exactly accounted for by the Na+ amounts present in the solid substrate.
The rate of ion uptake by roots was estimated by dividing the amount of ion absorbed per plant over the experiment duration to the mean root dry weight. The K+ net uptake rate was 58% lower, and much more inhibited by NaCl, in Columbia-0 than in NOK2 (Fig. 3). For plants grown on NaCl, the Na+ net uptake rate was 2 to 30 fold higher than the net K+ uptake. When K+ concentration in the culture medium was 0.1 mM, the estimated values of the Na+ net uptake rate were close to 5 mmol d−1 g−1 root DW for both Columbia-0 and NOK2. Increasing the K+ concentration from 0.1 mM to 2.5 mM resulted in a slight increase of the net Na+ uptake rate in Columbia-0 and a slight decrease in NOK2 (Fig. 3). In Columbia-0, net K+ uptake remained equally strongly inhibited by NaCl whatever the external K+ concentration, while in NOK2, virtually complete alleviation of the NaCl-induced inhibition of net K+ uptake was obtained by increasing the K+ concentration in the culture medium (Fig. 3). Altogether these results show that NOK2 was more efficient than Columbia-0 for K+ uptake, particularly in the presence of NaCl in the culture medium, and that treatment which increased K+ uptake also slowered Na+ build-up in NOK2 tissues.
3.3 Analyses of the gene expression of selected K+ and Na+ transporter
The gene expression of transporters involved in K+ and Na+ uptake and in K+ and Na+ transport between root and shoot was analysed in Columbia-0 and NOK2 plants grown on media supplemented with the already mentioned different combinations of K+ and NaCl concentrations. Semi quantitative RT-PCR analyses were done for the AKT1, AKT2 and SKOR K+ channels, and the NHX1 and SOS1 Na+/H+ antiporters. Since Ef-1α had already been demonstrated to show stable expression in plants submitted to varying K+ or Na+ external concentrations [17,29], it was used as an internal control. The mRNA abundances of NHX1 and SOS1 did not really change in response to the increase in the K+ concentration in the culture medium. They were however markedly increased in plants submitted to salt stress. SOS1 mRNA accumulation was 3 times augmented by NaCl, except in Columbia-0 shoots where this augmentation was only 2 to 2.5 times. Salt-induced NHX1 mRNA accumulation was essentially observed in the shoots. It was two fold higher in NOK2 plants than in Columbia-0 plants. The mRNA abundances of the three K+ channels were altered differently from each other in response to K+ supply and NaCl treatment. The AKT1 mRNA abundance in shoot remained the same, whatever the condition considered. AKT1 expression in roots was however strongly inhibited in response to NaCl stress, whatever the K+ concentration in the medium. Interestingly, the NaCl-induced reduction in AKT1 mRNA accumulation was slightly less pronounced in NOK2 roots ( reduction) than in Columbia-0 ones ( reduction). Almost no change in AKT2 and SKOR mRNA abundance was observed, except in NOK2 in response to 2.5 mM K+. Quite surprisingly, in that growing condition, AKT2 was induced but only in shoots, and SKOR was also induced, but only in roots. These responses were irrespective of the presence of NaCl in the culture medium, and were thus specific of the presence of a high K+ external supply.
In summary, the two Na+/H+ antiporters SOS1 and NHX1 and the AKT1 K+ channel displayed an alteration of their mRNA accumulation only in response to the NaCl treatment, being insensitive to changes in the K+ supply, while the AKT2 and SKOR K+ channels showed alteration of their mRNA accumulation only in response to the increasing K+ supply, being insensitive to the presence or absence of NaCl. There was a marked differential response between the two accessions for all genes: (i) increase in NHX1 mRNA accumulation in response to NaCl was greater in NOK2 than in Columbia-0; (ii) increase in SOS1 mRNA accumulation in response to NaCl was less pronounced in Columbia-0 shoots than in NOK2 ones; (iii) decrease in AKT1 mRNA accumulation in response to NaCl was slightly lower in NOK2 than in Columbia-0 and alteration of AKT; (iv) SKOR mRNA accumulation was only observed in NOK2.
4 Discussion
The comparison between the salt tolerant NOK2 and the salt sensitive Columbia-0 A. thaliana accessions revealed that NOK2 showed a higher salt tolerance than Columbia-0 that could be correlated to a greater K+ vs. Na+ selectivity [10]. Here we further analysed these two accessions and compared their performances in response to increasing K+ concentrations in the culture medium in presence vs. absence of a mild salt stress.
Our analyses confirmed that NOK2 is endowed with greater K+ vs. Na+ selectivity than Columbia-0 (Fig. 3E). The K+ content and the K+ net uptake rate were both higher in NOK2 than in Columbia-0 plants (Fig. 3), showing that NOK2 has an intrinsic high ability to absorb K+ compared to Columbia-0. Increasing K+ concentrations in the culture medium actually had a positive effect on growth in NOK2 but not in Columbia-0 (Fig. 1A). In response to the NaCl constraint, NOK2 plants showed less reduction in their growth and net K+ uptake compared to Columbia-0 plants (Figs. 1, 3). Using split root system approaches on glycophyte and halophyte species, several works have demonstrated that nutritional disturbances are implicated in the restriction of the growth under salinity stress [30–32]. Our molecular results showed that the difference in the AKT1 expression was not so great between the two accessions (Fig. 4), even if AKT1 is considered as a major contributor to K+ uptake from the soil solution [12,13,17]. This result is reminiscent of previous reports advancing that AKT1 is not regulated by changes in the K+ availability [12,33]. This suggests that K+ uptake is controlled at a different level or through the regulation of other(s) transporter(s). In contrast, a NOK2 specific up-regulation was observed for both the AKT2 and SKOR K+ channel genes at high K+ concentration (2.5 mM). This was observed for SKOR in the roots, and for AKT2 in the shoot. Indeed, these two K+ channels are involved in long distance transport of potassium, controlling the K+ loading into the xylem (SKOR) or into the phloem (AKT2) [18,19,34]. Over-expression of these two K+ channels might facilitate the translocation of K+ from the roots to the transpiring mature leaves (SKOR) and then the K+ circulation from the old leaves to the young ones (AKT2). This would contribute to raise the K+ content to high levels in shoots tissues, which characterizes NOK2 as compared to Columbia-0.
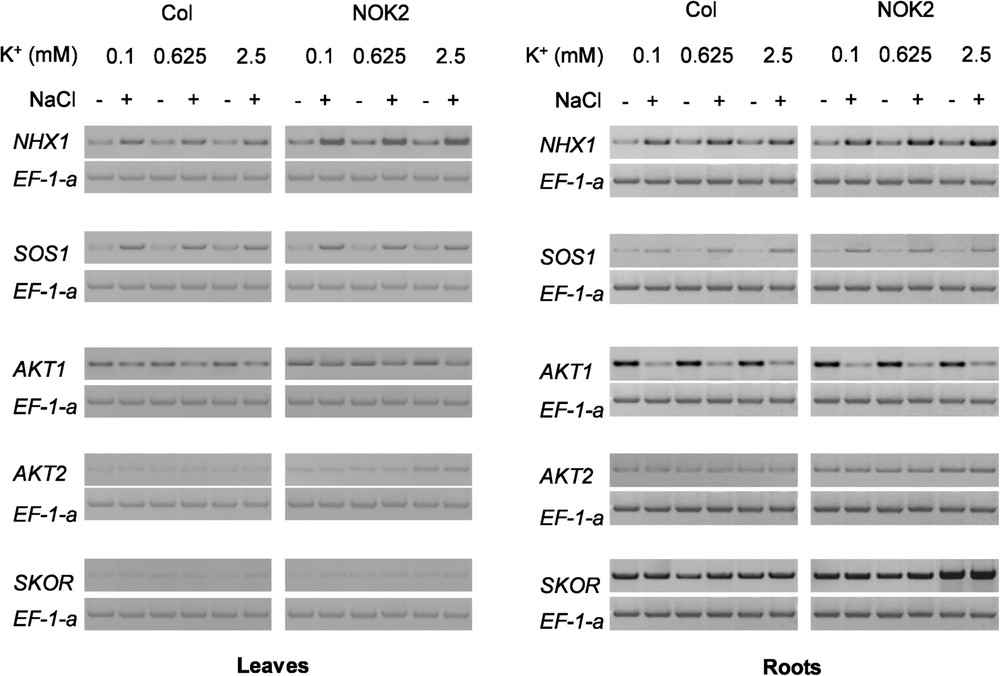
Semiquantitative RT-PCR analysis of NHX1, SOS1, AKT1, AKT2 and SKOR gene expression in NOK2 and Columbia-0 (Col) plants submitted to combined effects of increasing K+ concentration in the culture medium and applying or not a 50 mM NaCl treatment. The 0.1, 0.625 and 2.5 mM K+ and 0 or 50 mM NaCl treatments were applied to 23 day-old individual plants and lasted 17 days. Shoots and roots were analysed separately. Amplification conditions were as described in “Materials and Methods”.
The cause behind the higher salt tolerance of NOK2 in comparison to Columbia-0 was not found to derive from NOK2's greater ability to limit Na+ absorption by roots, since the net Na+ uptake was similar in the two accessions. The leaf Na+ content was however much higher in Columbia-0 than in NOK2 (Fig. 3). This could be the consequence of a lower expression of the SOS1 Na+/H+ antiporter in Columbia-0 shoots compared to NOK2 ones (Fig. 4). Indeed, SOS1 controls long distance Na+ translocation from root to shoot in Arabidopsis [26] and over-expression of SOS1 increases tolerance to NaCl stress [35]. However, SOS1 over-expression was probably not the main factor responsible for the reduction in leaf Na+ accumulation observed in NOK2 as compared to Columbia-0. The reduced Na+ content in NOK2 leaves was more likely a consequence of Na+ dilution in the larger leaf biomass in this accession. Indeed, NOK2 leaves accumulated more than twice less sodium than Columbia-0 ones and, in the same time, NOK2 plants were two and three times bigger than Columbia-0 ones (Figs. 1, 3). The observed dehydration of Columbia-0 leaves that is concomitant with Na+ accumulation (Figs. 2, 3) suggests that Na+ ions transported to these organs were not correctly compartmentalized in leaf cells. In contrast, the observation that Na+ accumulation was correlated with leaf succulence in NOK2 (Figs. 2, 3) suggested that Na+ ions imported in leaves participated in retaining water in leaf cells. This could be related to the fact that NHX1 was over-expressed in response to salt to a higher level in NOK2 than in Columbia-0 (Fig. 4). NHX1 is indeed responsible for compartmentalizing Na+ into the vacuole, which participates in maintaining cellular integrity in plants challenged by salt, and attenuates the salt sensitivity in Arabidopsis [22,36]. A greater Na+ storage in the vacuole could enable a greater increase in leaf hydration upon salt treatment in NOK2 plants, while a defect in this function would cause leaf dehydration and necrosis in salt-treated Columbia-0 plants, as it has been proposed for other glycophytes [37–41].
In summary, our results evidence differences in K+ and Na+ transports between NOK2 and Columbia-0, which could explain the difference in salt tolerance between the two accessions. NOK2 appears to display a greater cellular and tissular tolerance. This is evidenced by an increase in the water content, leaf thickness and ultimately growth, which discriminates NOK2 from Columbia-0. These phenotypic observations can be correlated with a greater transcript accumulation of the NHX1 tonoplastic Na+/H+ antiporter gene that would suggest that NOK2 efficiently compartmentalizes Na+ in its leaf cell vacuoles. However, the main characteristic discriminating NOK2 from Columbia-0 is its superior ability to take up K+ from the culture medium in the presence of NaCl. The molecular bases for this trait are still unknown, but the two K+ channels controlling long distance K+ transport in the plant (SKOR and AKT2) can be proposed to be candidates for explaining the NOK2 higher performances. Our results enlighten the usefulness of considering NOK2 as a crucial accession for further analysing K+ nutrition and K+ vs. Na+ transport at the molecular level.
Acknowledgements
This work was supported by a grant from Tunisian–French, CMCU (network 02F/924).